Inoculating rhizome-propagated Sporobolus pumilus with a native mycorrhizal fungus increases salt marsh plant growth and survival
Abstract
Salt marshes are ecosystems of significant ecological importance for coastal stability and fundamental roles in marine ecosystems. Salt marshes are declining due to anthropogenic and natural causes including sea level rise. Coastal restoration efforts have increased worldwide, but many fail in long-term coastal stability. We used a naturally occurring arbuscular mycorrhizal fungus (AMF) to test whether survival and early growth of the salt marsh grass Sporobolus pumilus (formerly Spartina patens) improved under simulated salt marsh conditions. Using a tidal mesocosm bench, we grew inoculated plants with varying AMF treatments under simulated tidal regimes to determine if AMF could aid in establishment of healthy Sporobolus communities. Rhizome-derived S. pumilus had greater survival and grew faster than seed-derived plants. Plants inoculated with propagated AMF consistently outperformed both sterile and native sediment controls in terms of plant survival and growth. Use of rhizome-derived Sporobolus inoculated with propagated Funneliformis geosporum showed the most promise in producing successful plant populations for salt marsh restoration. This may be due to plant life stage and improved plant nutrient status, allowing rhizome-derived plants to grow more quickly than seed-derived plants. Using these plants in future large-scale restoration may increase re-establishment of salt marsh ecosystems.
Introduction
Sporobolus (formerly Spartina) species have been used in Asia, Europe, and North America for salt marsh restoration, increasing bird and fisheries habitat, providing pasture for livestock, reclaiming lost salt marshes, and stabilising coastlines (Broome et al. 1988; Cooke and Lefor 1990; Warren et al. 2002). Sporobolus have extensive root networks that provide infrastructure and stability to salt marsh sediments (Broome et al. 1988). Sporobolus pumilus (Roth) is commonly used in salt marsh restoration, due to root branching traits and far-reaching rhizome growth (Shepard et al. 2011). Sporobolus pumilus has low seed germination rates; seeds are easily washed away by tidal forces, making seedling transplants the method of choice for restoration (Cooke and Lefor 1990). Little is known about seed predators of this species, which may further decrease the chance of S. pumilus survival using restorative seed stock. In the Bay of Fundy region of the Canadian Maritimes, mega-tidal dynamics and winter ice scouring compound these challenges. Successful restoration is difficult and needed to provide long-term functioning and conservation of salt marsh ecosystems (Callaway 2005).
Re-examination of salt marsh sites 10 years postrestoration reveals that restored areas can revert back to tidal flats, even when seedling transplants were used. Tissue analysis from native and restored S. pumilus indicated the restored plants were not forming symbioses with arbuscular mycorrhizal fungi (AMF), which restricted establishment and growth (Cooke and Lefor 1990). AMF affect the competitive ability of salt marsh plants by increasing access to sediment phosphorous and nitrogen (Daleo et al. 2008). The inability to re-establish the mutualism between S. pumilus and AMF may point to the absence of AMF in infilled sediments, which our project works to identify and remedy. In Nova Scotia (NS), Canada, salt marsh restoration practices have been successful after dyke breaches and culvert expansion, with 100% vegetation cover (primarily Sporobolus alterniflorus (Loisel.)) 3 years postbreach, but these projects have not examined local AMF (Bowron et al. 2012; van Proosdij et al. 2010); long-term plant survival is unknown.
We previously isolated and identified the cosmopolitan halotolerant AMF species Funneliformis geosporum (T.H. Nicolson & Gerd.) (Hildebrandt et al. 2001; Landwehr et al. 2002) from a salt marsh in Wolfville, NS; this obligately symbiotic fungus may be of critical importance to S. pumilus establishment and growth (d’Entremont et al. 2018). Funneliformis geosporum may produce 80% of all AMF spores found in salt marshes (Hildebrandt et al. 2001). Currently, F. geosporum remains understudied, but likely plays a crucial role in the Sporobolus root endophytic community in the salt marshes of Wolfville Harbour (d’Entremont et al. 2018). Use of this obligate mutualistic fungus in simulated salt marshes may reveal the importance of this species to salt marsh ecosystem structure. Controlling environmental parameters, such as light intensity and tidal inundation, while still producing seminatural conditions may provide evidence to support the role of F. geosporum (Callaway et al. 1997). Mesocosms are useful tools for testing factors and restoration techniques prior to large-scale implementation, offering great potential for evaluation (Callaway et al. 1997).
The objectives of this study were to: (i) identify whether inoculation of S. pumilus with F. geosporum increased survival and growth, compared with a sterile control, under simulated salt marsh conditions (tidal mesocosm); (ii) determine if F. geosporum inoculant outperformed the use of natural sediment in improving survival and growth of S. pumilus under simulated salt marsh conditions; and (iii) determine whether AMF-inoculated rhizome- or seed-derived S. pumilus survived better under simulated salt marsh conditions. We predicted that using rhizome-propagated plants inoculated with F. geosporum would outperform all other treatments under our simulated salt marsh conditions due to plant life stage and increased AMF colonisation.
Materials and methods
Seed processing
Sporobolus pumilus stalks and seed were collected on 20 September 2017 from Wolfville Harbour, NS, salt marsh (Fig. S1; Google Earth 2020) and stored in paper bags. Seeds were processed after air drying (7 d at room temperature). Final storage was at 4 oC in the seed collection at the E.C. Smith Herbarium, Acadia University, Wolfville, NS.
Arbuscular mycorrhizal fungi propagation
The fungus (F. geosporum) was propagated in 12 trap pots in the K.C. Irving Environmental Science Centre greenhouse at Acadia University (Wolfville, NS). Plastic trap pots (2 L) contained a homogenous mixture of autoclaved sand and natural salt marsh sediment (Morton et al. 1993). Ten untreated corn seeds (Zea mays; Vesey Seeds) were planted and grown using groundwater sparingly to create water stress (no nutrient addition). Only five corn seedlings were kept (the first to emerge), with the other seedlings being removed to prevent overcrowding. Temperature was held at 23 °C ± 2°C under a 14-h lighting regime (approx. 500 μmolm−2s−1).
Eight weeks postinitiation, corn plants were sacrificed by cutting the stem at soil surface level. Moisture was removed from inoculant for 1 week at room temperature to initiate sporulation. Quality of inoculant was assessed by observing AMF colonisation of each corn plant prior to storage, and AMF spore counts of inoculant were performed before the initiation of each trial. AMF colonization was observed using root clearing and staining of each corn plant and was averaged since the inoculant from each pot was combined into a common batch (μ = 72%, n = 60) (Vierheilig et al. 1998). The inoculant was stored covered at 4 °C until needed for each trial. AMF spores were counted in triplicate using sieving and glucose gradient centrifugation prior to use (8 spores per gram, n = 3) (Morton et al. 1993).
Sporobolus pumilus initiations
From rhizome fragments
Rhizome fragments (2–3 cm) of Sporobolus pumilus, collected from Wolfville Harbour salt marsh (16 August 2017), were rinsed with reverse osmosis (RO) water in metal mesh tea balls (mesh size <500μm) then placed under running tap water for 1 h. Fragments were transferred to 50% isopropanol for 30 s, rinsed with sterile RO water, then transferred to 1% sodium hypochlorite with 2 drops of Tween 80. The vessels were agitated for 10 min, poured off, and rinsed 4 times with RO water inside a laminar flow bench. The fragments were then transferred to media containing 10 mL/L Plant Preservative Mixture (PPM) + ½ Murashige and Skoog (MS) + Ampicillin (A) plates for 3 d, then 1.0 mL/L PPM + ½ MS + A plates for 1–2 weeks. Fragments were then moved to ½ MS + A medium in 25 mm × 90 mm glass vials and later to Magenta vessels (77 mm length × 77 mm width × 97 mm height). These populations were maintained on a 14-h lighting regime at 20 °C, in a dark room, until needed for the growth trials to ensure sterility. Periodic sectioning of the plants kept them small and manageable within the Magenta vessels.
From seed
Local seeds of Sporobolus pumilus from the E.C. Smith Herbarium, were placed on moist paper towel for 24 h prior to sterilization. Seeds were sterilized using the same technique as rhizome fragments except seeds were agitated in sodium hypochlorite and Tween 80 for 15 min instead of 10 min, rinsed, and transferred directly to ½ MS + A in 100 × 15 Petri dish after the 10 mL/L PPM + ½ MS +A treatment.
Growth trial inoculations
Sterile rhizome fragments were sectioned into two pieces (∼2 cm) with a sterile scalpel on multiple occasions to control the growth of the plants and ensure they would not be too large for the growth trials. Seedlings were grown on ½ MS + A media in Petri dishes for 10 days prior to exposure to the fungal inoculant. It was deemed appropriate using a power of t-test, that 30 plants per test group would be sufficient, per group, to detect differences between the populations (δ = 0.73). Each plant was assigned a number and its respective group was chosen via a random number function in Microsoft Excel to reduce bias.
Simulated salt marsh trial 1: rhizome-derived plants, sterile sand (proof of concept)
Forty-eight rhizome-derived S. pumilus were planted in a 1:1 mixture of autoclaved sand and trap pot propagated F. geosporum inoculant (8 spores per gram) in a potting tray (26 cm width × 52 cm length × 7 cm deep) on 7 May 2018. The 48-rhizome-derived S. pumilus controls were planted in autoclaved sand. Plants were grown for 21 d under greenhouse conditions with a 14-h lighting regime, daily moisture monitoring, and temperature control at 23°C ± 2°C. All plants were assessed for AMF colonisation at the end of the experimental trial, showing an average colonisation of 64% (n = 48) for inoculated and 0% (n = 48) for control (Vierheilig et al. 1998).
Simulated salt marsh trial 2: rhizome-derived plants, natural salt marsh sediment
Forty-two S. pumilus plants were inoculated using a 1:1 mixture of trap pot propagated F. geosporum inoculant (8 spores per gram) and natural salt marsh sediment on 1 June 2018. Control plants were planted in 1:1 mixture of autoclaved sand and natural salt marsh sediment. Plants were grown as above with an average AMF colonisation of 17% (n = 42) for plants inoculated with propagated F. geosporum and 9% (n = 42) for natural sediment control plants (Vierheilig et al. 1998).
Simulated salt marsh trial 3: seedlings, natural salt marsh sediment
Each treatment contained 30 plants grown either using a 1:1 mixture of trap pot propagated F. geosporum inoculant (8 spores per gram) and natural salt marsh sediment, or a control (1:1 mixture of autoclaved sand and natural salt marsh sediment). Plants were grown as described previously and were initiated on 9 October 2018, with an average AMF colonisation rate of 7% (n = 30) for plants inoculated with propagated F. geosporum, and 3% (n = 30) for control plants (natural sediment) (Vierheilig et al. 1998).
Mesocosm growth trial
Groundwater was used to dilute 1200 L of seawater, obtained from the Minas Basin, NS, to a salinity range of 18–22 ppt. All experiments were performed in a tidal mesocosm bench using a natural tidal cycle, to match the Minas Basin and create natural inundation of the plants by tidal waters. The mesocosm bench was custom designed by Groupe DHB Inc. for the K.C. Irving Environmental Science Centre (Wolfville, NS). Dimensions of the stainless-steel bench are: 181 cm wide, 312 cm long, and 45 cm deep (Fig. 1). The tidal program consisted of a 3 h flood tide followed by a 3 h ebb tide and a hold at low tide for 6 h 28 min, to advance the tide each cycle. Tides were simulated using a peristaltic pump for high tide or a dump valve for low tide.
Fig. 1.

Natural salt marsh sediment (collected from Wolfville Harbour) was used in custom 1.5 cm thick plexiglass pots (10 cm diameter, 10 cm height). The bottom of the pipe was sealed with Nitex (75 μm) mesh and a plastic grill to facilitate water exchange without losing sediment (Haskett 2018). Plexiglass racks were built to suspend the pots 6 cm above the bottom of the bench, with the maximum inundation of the cores (at high tide) covering the cores (< 1 cm).
A 14-h photoperiod was used to increase the light exposure to approximately 500 μmolm−2s−1. Salinity was monitored daily using an Oakton Salt 6+ handheld salinity probe and adjusted using groundwater. Shoot length and survival of plants was monitored weekly throughout the 2018 experiment duration until one treatment had reached approximately 50% mortality. At the end of each trial, all plants were sacrificed for shoot length measurements to determine whether the effects of AMF inoculation on the growth of the S. pumilus plants were statistically significant.
Statistical analysis
Shoot length
A Welch’s two-sample t-test (α = 0.05) was used to compare shoot length of S. pumilus in the two treatment groups after the 21-d treatment period (prior to entering the tidal mesocosm bench) as well as postexperimental trial. Shoot length was measured from the soil level to the tip of the shoot, without removing plants from their treatment.
Shoot length growth
For each plant, the final shoot length (postgrowth trial) was subtracted from the initial shoot length (measured after the experimental treatment), to determine the average growth for each experimental group (F. geosporum inoculated or control). We used a Welch’s two-sample t-test (α = 0.05) to compare inoculated and control groups to determine if relative increase in shoot length was significantly different.
Results
Simulated salt marsh trial 1: rhizome-derived plants, sterile sand (proof of concept) (n = 96)
Growth of S. pumilus was monitored for 42 d, between 7 May 2018 and 18 June 2018, with AMF-inoculated plants surviving better under simulated salt marsh conditions over that period (85% survival compared to 44%) (Fig. 2a). Shoot lengths of AMF-inoculated S. pumilus were significantly longer than the controls immediately after the 21-d treatment period (t(85) = 12.17, p < 0.001) and overall after 42 d (t(55.36) = 14.05, p < 0.001) (Fig. 2b). The average shoot length increase over the period was also significantly greater for the AMF-inoculated group (t(44.90) = 2.18, p = 0.034) (Fig. 2b).
Fig. 2.

Simulated salt marsh trial 2: rhizome-derived plants, natural salt marsh sediment (n = 84)
Plants were monitored for 48 d, between 22 June 2018 and 9 August 2018, with higher survival observed for the AMF-inoculated group under simulated salt marsh conditions (90% survival compared to 57%) (Fig. 3a). Shoot length of AMF-inoculated S. pumilus was significantly longer than the control plants after the 21-d treatment period (t(79.65) = 2.99, p = 0.004) and overall (t(54.92) = 2.92, p = 0.005) (Fig. 3b). Shoot growth was not significantly different (t(59.87) = 0.53, p = 0.596) (Fig. 3b).
Fig. 3.
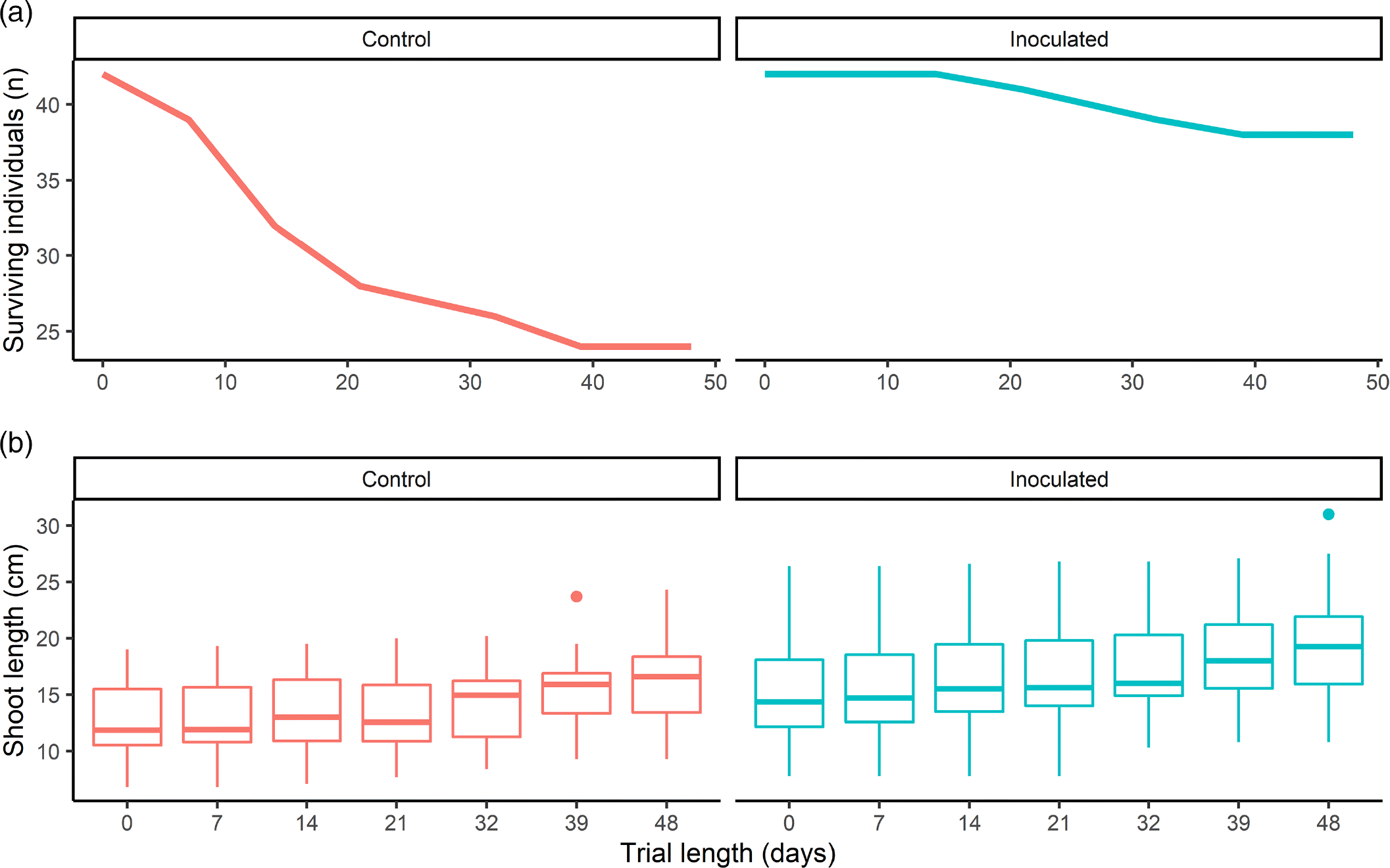
Simulated salt marsh trial 3: seedlings, natural salt marsh sediment (n = 60)
Sporobolus pumilus seedlings were grown for 27 d, between 30 October 2018 and 26 November 2018, with AMF-inoculated seedlings showing higher survival than seedlings grown in the natural sediment control (63% vs. 40%) (Fig. 4a). Shoot lengths of AMF-inoculated S. pumilus were not significantly different than the control plants after the 21-d treatment period (t(49.78) =1.68, p = 0.099), nor were they different after the 27-d growth trial (t(27.30) = 1.28, p = 0.21) (Fig 4b). Shoot growth was significantly higher for the inoculated group (t(26.61) = 2.52, p = 0.018) (Fig. 4b).
Fig. 4.

Table 1 summarizes the data from each of the salt marsh trials.
Table 1.
Mean ± C.I. length (cm) | ||||||
---|---|---|---|---|---|---|
Trial no. | Treatment | t0 | t1 | Δ | AMF, t0 | Survival, t1 |
1 | Inoculated | 25.9 ± 10.5 | 29.8 ± 12.7 | 3.7 ± 0.1 | 64% | 85% |
Control | 13.3 ± 14.6 | 15.1 ± 16.9 | 2.4 ± 2.5 | 0% | 44% | |
2 | Inoculated | 14.9 ± 0.8 | 19.4 ± 1.0 | 4.2 ± 1.1 | 17% | 90% |
Control | 12.5 ± 4.1 | 16.3 ± 5.2 | 3.7 ± 1.9 | 9% | 57% | |
3 | Inoculated | 16.5 ± 0.3 | 18.2 ± 1.0 | 2.1 ± 0.3 | 7% | 63% |
Control | 14.9 ± 3.4 | 16.6 ± 4.3 | 0.8 ± 2.44 | 3% | 40% |
Discussion
Simulated salt marsh trial 1: rhizome-derived plants, sterile sand (proof of concept)
Survival of AMF trap-pot inoculated S. pumilus was much higher than the control, indicating that F. geosporum may help offset the shock of the highly saline salt marsh conditions for these plants. Previous research has shown improved salt stress tolerance by plants when colonized by AMF (Giri et al. 2007). Early survival is fundamental to healthy plant community establishment and sustainability (Cooke and Lefor 1990); higher early survival reduces the planting density needed for S. pumilus in salt marsh restoration projects, saving both time and money. Our trial also demonstrated that inoculation increased the growth and final length of S. pumilus under simulated salt marsh conditions, which are also factors that contribute to the successful establishment of salt marsh ecosystems; healthy vegetation slows tidal waters, leading to increased sedimentation and salt marsh growth (Fagherazzi et al. 2006).
We also found a significant growth difference between the AMF-inoculated S. pumilus plants and the uninoculated control group after the 21-d treatment period. Evidence of AMF influence was already present, with significantly greater shoot lengths prior to exposure to simulated salt marsh conditions in our tidal mesocosm. Natural salt marsh sediment contains nutrients that may not be present in autoclaved sand, which may have aided in growth along with the AMF, although the chemical composition was not tested for either treatment. Autoclaving sand increases the nutrient availability of the substrate (Badía and Martí 2003), but we expect the nutrients provided by the natural salt marsh sediment inoculant to be greater even though the nutrient profile of the sand may have been positively altered during autoclaving. For this reason, our control group was biased but served as a proof of concept trial, showing us that the inoculant, whether it be nutrients or AMF, does improve growth over no treatment at all. Conversely, the salt marsh sediment also introduces possible stressors such as salts (and potentially pathogens), which are naturally present in the sediment used to create the inoculant. The AMF present in the inoculant may remedy the increase in salt, while maintaining the benefits of the added mineral nutrients; if this is occurring, the mechanism remains unknown (Estrada et al. 2013). Our results show AMF inoculant significantly increased the size of the treated S. pumilus, which increased their survival and growth under simulated salt marsh conditions.
Simulated salt marsh trial 2: rhizome-derived plants, natural salt marsh sediment
This trial compared our AMF inoculant against natural salt marsh sediment containing naturally occuring nutrients and microorganisms surrounding S. pumilus roots. By using this as a treatment, we can assess whether our inoculant outperforms natural sediment, where S. pumilus would naturally grow. Mixed natural sediment and autoclaved sand (in a 1:1 ratio) were used to give the control treatment the same texture as the AMF inoculant treatment. Sediment characteristics, including porosity (Martin et al. 2012), may play a role in the colonisation of S. pumilus roots by AMF; therefore, similar sediment types were sought in our comparison of natural sediment against and propagated AMF inoculant.
As in mesocosm trial 1, the survival of S. pumilus inoculated with our AMF propagated treatment was higher than the natural sediment control (90% vs. 57%, n = 84).These treatments differed only regarding fungal load, indicating that AMF may be the driver of this survival. Shoot lengths of AMF-inoculated S. pumilus were significantly higher than the control group despite similar sediment treatments and lower average AMF colonisation than the previous trial (17% for the inoculated and 9% for the control, n = 84). The significantly larger size of inoculated S. pumilus persisted throughout the 48-d mesocosm trial, although the overall length increase of the two groups did not differ significantly. This experiment indicates that using a propagated inoculant may be beneficial, rather than rearing S. pumilus in natural sediment, for early plant establishment in salt marsh restoration.
Simulated salt marsh trial 3: seedlings, natural salt marsh sediment
In this trial we tested S. pumilus seedlings as opposed to rhizome-derived plants (trials 1 and 2). Conditions of the second experiment were repeated to determine if the same trends would be found for seedlings. Although Nova Scotian salt marsh restoration projects have not focused on active planting, many salt marsh restoration projects elsewhere have focused on transplanting S. pumilus propagated from collected seed stock due to its ease and low cost; many of these projects failed (Cooke and Lefor 1990). Thus, a comparison between seedlings and rhizome-derived plants was completed to determine if one method of salt marsh plant propagation may be superior, for restoration purposes.
The AMF-inoculated group had higher survival than the control group, even though the average AMF colonisation, prior to the mesocosm growth trial, was low for both groups (7% and 3% respectively, n = 60, as assessed 21-days after treatment). Trial 3 duration was only 27 d, due to the rapid death of both S. pumilus test groups. There was no difference in S. pumilus size after the 21-d inoculation period nor the 27-d mesocosm growth trial. Interestingly, although the groups didn’t differ in length, the inoculated plants grew significantly more than the control, when looking at their final size in comparison to their initial size. In many ways, the AMF-inoculated plants outperformed the control groups in all three of our trials.
Comparison of the survival of seedlings (trial 3) to the rhizome-derived S. pumilus (used in trials 1 and 2) showed that rhizome-derived plants had better survival rates, possibly due to differences in the AMF colonisation abilities of adult tissue to juvenile tissue, although this was not tested. Previous research on the seagrass (submerged angiosperm) Cymodocea nodosa showed that vegetative progeny from adult clones had a better chance of substrate colonisation than seedling C. nodosa (Balestri and Lardicci 2012). These results are mirrored by our experiment which showed higher survival for rhizome-derived S. pumilus, indicating that future restoration projects using S. pumilus should use AMF-inoculated rhizome-derived plants.
Conclusion
Collectively these three salt marsh mesocosm growth trials demonstrated that survival was consistently lower for non-AMF-inoculated Sporobolus pumilus plants. Sporobolus pumilus rhizome treated with F. geosporum inoculant had higher survival and grew larger than the control plants. Comparison of seedling survival (trial 3) to the trial 1 and 2 rhizome-derived S. pumilus plants demonstrated greater survival for rhizome-derived plants, indicating this source material may be preferable to seedlings for salt marsh restoration. These findings, taken together, provide guidance for ongoing and future salt marsh restoration in Atlantic Canada of critical interest due to climate change.
Acknowledgements
T.W. d’Entremont thanks the Arthur Irving Academy for the Environment for a Master’s Student Scholarship in Environmental Science. A.K. Walker and J.C. López-Gutiérrez gratefully acknowledge an Arthur Irving Academy Research Grant to A. Walker, J.C. López-Gutiérrez and R. Browne. AKW also acknowledges funding from an NSERC Discovery Grant (No. NSERC—2017-04325). All authors extend our thanks to the K.C. Irving Environmental Science Centre staff D. Kristie, R. Browne, and J. Walker along with G. Gibson (Acadia University Department of Biology).
References
Badía D, and Martí C. 2003. Plant ash and heat intensity effects on chemicaland physical properties of two contrasting soils. Arid Land Research and Management, 17: 23–41.
Balestri E., and Lardicci C. 2012. Nursery-propagated plants from seed: a novel tool to improve the effectiveness and sustainability of seagrass restoration. Journal of Applied Ecology, 49: 1426–1435.
Bowron TM, Neatt N, van Proosdij D, and Lundholm J. 2012. Salt marsh tidal restoration in Canada’s Maritime provinces. In Tidal Marsh Restoration. Edited by CT. Roman and DM Burdick Island Press/Center for Resource Economics, Washington, DC, pp. 191–209.
Broome SW, Seneca ED, and Woodhouse WW. 1988. Tidal salt marsh restoration. Aquatic Botany, 32: 1–22.
Callaway JC. 2005. The challenge of restoring functioning salt marsh ecosystem. Journal of Coastal Research, 24–36.
Callaway JC, Zedler JB, and Ross DL. 1997. Using tidal salt marsh mesocosms to aid wetland restoration. Restoration Ecology, 5: 135–146.
Cooke JC, and Lefor MW. 1990. Comparison of vesicular-arbuscular mycorrhizae in plants from disturbed and adjacent undisturbed regions of a coastal salt marsh in Clinton, Connecticut, USA. Environmental Management, 14: 131–137.
d’Entremont TW, López-Gutiérrez JC, and Walker AK. 2018. Examining arbuscular mycorrhizal fungi in saltmarsh hay (Spartina patens) and smooth cordgrass (Spartina alterniflora) in the Minas Basin, Nova Scotia. Northeastern Naturalist, 25: 72–86.
Daleo P, Alberti J, Canepuccia A, Escapa M, Fanjul E, Silliman BR, et al. 2008. Mycorrhizal fungi determine salt-marsh plant zonation depending on nutrient supply. Journal of Ecology, 96: 431–437.
Estrada B, Aroca R, Barea JM, and Ruiz-Lozano JM. 2013. Native arbuscular mycorrhizal fungi isolated from a saline habitat improved maize antioxidant systems and plant tolerance to salinity. Plant Science, 201–202: 42–51.
Fagherazzi S, Carniello L, D’Alpaos L, and Defina A. 2006. Critical bifurcation of shallow microtidal landforms in tidal flats and salt marshes. Proceedings of the National Academy of Sciences of the United States of America, 103: 8337–8341.
Google Earth. 2020. Wolfville Harbour salt marsh. [online]: Available from google.com/earth/index.html.
Giri B, Kapoor R, and Mukerji KG. 2007. Improved tolerance of Acacia nilotica to salt stress by arbuscular mycorrhiza, Glomus fasciculatum may be partly related to elevated K/Na ratios in root and shoot tissues. Microbial Ecology, 54: 753–760.
Haskett EC. 2018. Response of intertidal benthic invertebrate assemblages to high and low concentrations of suspended sediment. Acadia University thesis.
Hildebrandt U, Janetta K, Ouziad F, Renne B, Nawrath K, and Bothe H. 2001. Arbuscular mycorrhizal colonization of halophytes in Central European salt marshes. Mycorrhiza, 10: 175–183.
Landwehr M, Hildebrandt U, Wilde P, Nawrath K, Tóth T, Biró B, and Bothe H. 2002. The arbuscular mycorrhizal fungus Glomus geosporum in European saline, sodic and gypsum soils. Mycorrhiza, 12: 199–211.
Martin SL, Mooney SJ, Dickinson MJ, and West HM. 2012. The effects of simultaneous root colonisation by three Glomus species on soil pore characteristics. Soil Biology and Biochemistry, 49: 167–173.
Morton JB, Bentevenga SP, and Wheeler WW. 1993. Germ plasm in the international collection of arbuscular and vesicular-arbuscular mycorrhizal fungi (INVAM) and procedures for culture development, documentation and storage. Mycotaxon, 48: 491–528.
Shepard CC, Crain CM, and Beck MW. 2011. The protective role of coastal marshes: a systematic review and meta-analysis. PLoS ONE, 6: e27374.
van Proosdij D, Lundholm J, Neatt N, Bowron T, and Graham J. 2010. Ecological re-engineering of a freshwater impoundment for salt marsh restoration in a hypertidal system. Ecological Engineering, 36: 1314–1332.
Vierheilig H, Coughlan AP, Wyss U, and Piché Y. 1998. Ink and vinegar, a simple staining technique for arbuscular-mycorrhizal fungi. Applied and Environmental Microbiology, 64: 5004–5007.
Warren RS, Fell PE, Rozsa R, Brawley AH, Orsted AC, Olson ET, et al. 2002. Salt marsh restoration in connecticut: 20 years of science and management. Restoration Ecology, 10: 497–513.
Supplementary material
Supplementary Material 1 (DOCX / 781 KB)
- Download
- 780.52 KB
Information & Authors
Information
Published In
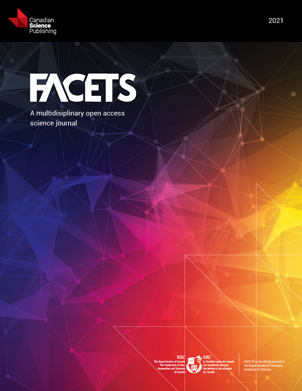
FACETS
Volume 6 • Number 1 • January 2021
Pages: 1134 - 1145
Editor: Iain E.P. Taylor
History
Received: 16 November 2020
Accepted: 12 February 2021
Version of record online: 8 July 2021
Copyright
© 2021 d’Entremont et al. This work is licensed under a Creative Commons Attribution 4.0 International License (CC BY 4.0), which permits unrestricted use, distribution, and reproduction in any medium, provided the original author(s) and source are credited.
Data Availability Statement
All relevant data are within the paper and in the Supplementary Material.
Key Words
Sections
Subjects
Plain Language Summary
Beneficial root fungi help salt marsh plants grow
Authors
Author Contributions
All conceived and designed the study.
TWD performed the experiments/collected the data.
All analyzed and interpreted the data.
JCL-G and AKW contributed resources.
All drafted or revised the manuscript.
Competing Interests
The authors have declared that no competing interests exist.
Metrics & Citations
Metrics
Other Metrics
Citations
Cite As
Tyler W. d’Entremont, Juan C. López-Gutiérrez, and Allison K. Walker. 2021. Inoculating rhizome-propagated Sporobolus pumilus with a native mycorrhizal fungus increases salt marsh plant growth and survival. FACETS.
6: 1134-1145.
https://doi.org/10.1139/facets-2020-0104
Export Citations
If you have the appropriate software installed, you can download article citation data to the citation manager of your choice. Simply select your manager software from the list below and click Download.
Cited by
1. Specificity in plant-mycorrhizal fungal relationships: prevalence, parameterization, and prospects