Why the English–Wabigoon river system is still polluted by mercury 57 years after its contamination
Abstract
Between 1962 and 1969, 10 tonnes of mercury were discharged from a chlor-alkali plant in Dryden, Ontario, to the English–Wabigoon River. Present-day fish mercury concentrations are amongst the highest recorded in Canada. In 2017, the Grassy Narrows Science Team found no evidence of ongoing discharges from the plant site to the river water, even though large quantities of mercury remain at the site. Instead, our data suggest that ongoing erosion of high mercury particles by the river, as it meanders through contaminated floodplains, is responsible for present-day transport of mercury to Clay Lake and to Ball Lake, located 154 km downstream. In Clay Lake, surface sediment total mercury concentrations and inflow water concentrations are still about 15 times above background (86 km downstream), and in Ball Lake mercury concentrations in sediments appeared to be still increasing. The remobilization of legacy inorganic mercury from riverbank erosion between Dryden and Clay Lake stimulates methyl mercury production there, in Clay Lake, and in Ball Lake. The large quantities of methyl mercury produced between Dryden and Clay Lake are mostly dissolved in water and are swept downstream, elevating concentrations in water and biota throughout the system. Several options for remediating the ongoing contamination are discussed.
Introduction
Between 1962 and 1969 the Dryden Chemical Company located in Dryden, northwestern Ontario, discharged approximately 10 tonnes of mercury from a chlor-alkali plant into the Wabigoon River (Fig. 1; Armstrong and Hamilton 1973). In 1974, mercury contamination of this river and lake system was found in fish as far as Tetu Lake, 250 km downstream of Dryden (Neff et al. 2012). During 1972 in the east basin of Clay Lake, which is the first lake (86 km) downstream of Dryden, surface sediment and water mercury concentrations were >6000 ng/g and 120 ng/L, respectively (Armstrong and Hamilton 1973). Fish mercury concentrations in axial muscle were up to 16 ppm wet weight (Armstrong and Hamilton 1973).
Fig. 1.

Methyl mercury (MeHg) is a potent human toxin (Mergler et al. 2007). Before the discharge of mercury to the Wabigoon, there was an active commercial and subsistence fishery operated by the Asubpeeschoseewagong Netum Anishinabek (Grassy Narrows) First Nation, with nearly 100% employment of the Grassy Narrows community, primarily at a fly-in fishing lodge located on Ball Lake (Fig. 1; Hutchinson and Wallace 1977). After the mercury contamination was discovered, the commercial fishing stopped. Some subsistence fishing continued, but at a much-reduced rate. The fishing lodge was closed, resulting in widespread social disruption of the community (Vecsey 1987). In addition to the loss of food and employment there was also severe MeHg poisoning of the Grassy Narrows people (Mosa and Duffin 2017).
The commercial fishery and lodge at Ball Lake remain closed today, and health effects of mercury contamination and social disruption are still evident in the Grassy Narrows community (Harada et al. 2011). Also, a recent study has found that premature mortality in the Grassy Narrows community is related to mercury concentrations in hair samples (Philbert et al. 2020).
Even though fish mercury concentrations in Clay Lake are amongst the highest measured in Canada (Fisheries and Oceans Canada 2021; Depew et al. 2013), there has been no systematic investigation of the mercury concentrations in water, sediments, and fish since a joint federal–provincial study of the system in the 1980s (Rudd et al. 1983; Parks et al. 1986). These 1980s studies resulted in a recommendation to add clean lacustrine clay particles to remediate the English–Wabigoon system (Rudd et al. 1983; Parks and Hamilton 1987). This solution, with a pilot study being the first step, was recommended by the Ontario Ministry of the Environment to the Cabinet of the time, but the cabinet instead opted to rely on natural recovery of the system, which has not yet occurred.
More recent studies of sediment and fish mercury concentrations in Clay Lake (Lockhart et al. 2000; Kinghorn et al. 2007; Neff et al. 2012) found that recovery was initially rapid for both sediments and fish. However, since the early 1980s, sediments have declined much more slowly and walleye mercury concentrations in muscle have plateaued at about 2 μg/g, wet weight, 4- and 10-fold higher than commercial and subsistence consumption guidelines, respectively. There were some measurements of mercury discharges from the plant site in the late 1970s after the chlor-alkali plant had closed (10–14 kg/year; Parks et al.1986), but no known discharge estimates have been made since then.
The objective of this study is to determine why total mercury (THg) concentrations in sediments were still so high (35 times above background in Clay Lake, Lockhart et al. 2000), and walleye wet weight in muscle MeHg concentrations are still 10 times above subsistence levels. There are two possibilities: mercury may be continuing to leak out of the former chlor-alkali site from contaminated groundwater or abandoned on-site mercury storage, and (or) there may be downstream inputs to the system from contaminated floodplains that are being remobilized and transported downstream. Because MeHg production and concentrations in mercury contaminated ecosystems are positively related to THg concentrations (Rudd et al. 2018), our study was designed to distinguish between these two possible inputs of THg, either one of which would support elevated MeHg production in the system.
Methods
Partnership
This study was done in partnership with the Grassy Narrows community. The Chief and Council of Grassy Narrows approved our study. For two decades, Judy DaSilva, the Grassy Narrows Environmental Health Co-ordinator, worked closely with Wiikogekwe (Patricia Sellers) on environmental aspects of the mercury pollution problem on the English–Wabigoon River. The Grassy Narrows Science Team was brought together by them in 2016, with the purpose of merging scientific investigation with Indigenous knowledge and concerns. A group of community members who are Traditional Knowledge holders about the river, The Grassy Narrows River Team, participated in field sampling and provided important input and feedback to the interpretation of the 2017 field results. The Science Team met with the community four times during the field season, to present and discuss results as they were obtained.
Site description
The Wabigoon River originates at the outflow of Wabigoon Lake, in the Wabigoon Basin, a Quaternary clay-rich basin on the Canadian Shield, northwestern Ontario. The basin sediments were deposited about 10,000 years ago, along the margin of the Rainy Lobe of the Laurentide Ice Sheet, which formed the northern boundary of proglacial Lake Agassiz (Sharpe et al. 1992). These glacial deposits are constantly being eroded from the shoreline of Wabigoon Lake, transporting fine clay material into Wabigoon Lake and then downstream to the Wabigoon River and throughout the system (Fig. 1, Fig. S7). This clay material is important to both the downstream transport and possible remediation of the English—Wabigoon system (see later discussions).
In this paper river kilometer (rkm) 0 is the origin of the Wabigoon River at the outflow of Wabigoon Lake (Fig. 1). The site of the former chlor-alkali plant is on the east bank of the Wabigoon River between rkm 1 to 4 (Fig. 1).
On 5 occasions during 2017 we surveyed the river water at 11 stations between Wabigoon Lake (rkm 0) and Ball Lake outflow (rkm 154, Fig. 1). The first and second surveys (8 May – 17 May and 23 May – 1 June) occurred over 9-day periods. The last three surveys were over shorter time periods (2–3 days). The first survey began 2 days after ice-off on Clay Lake and Ball Lake (8 May 2017). The last survey (27 September 2017) was about 2 weeks prior to lake freeze-up. Flows varied substantially during the sampling season with peaks in river flow at the spring freshet and twice during June and July (Fig. S9). Flows were substantially lower during August and September. The flow volumes and timing pattern, at the Water Survey of Canada Station (Fig. 1), were quite similar in 2017 to the 10-year average flow of the Wabigoon River (Fig. S11).
Clay Lake has a surface area of 3000 ha, a 20 m deep stratified western basin and a shallow unstratified eastern basin. Its theoretical water residence time is 57 days at an annual mean inflow of 57 m3/s. During the spring freshet, water residence times are on average 31 days (Parks and Hamilton 1987). Ball Lake has a surface area of 2700 ha and a maximum depth of 20 m. It has two inflows; the English River, which is the larger inflow, enters the north basin of Ball Lake (Fig. 1). The north basin has a mean annual water residence time of 5 days. The Wabigoon River enters the south basin of Ball Lake, which is an unstratified mixing zone with a water residence time of about half the north basin. The large inflow from the English River has chiefly prevented mercury contamination of the north basin of Ball Lake (Parks and Hamilton 1987).
Sample collection
Water samples for THg and MeHg analyses were collected using trace-metal clean techniques as described in St. Louis et al. (1994).
Samples for total suspended solids (TSS), inorganic particles (suspended), chloride (Cl−), sulfate (SO42−), and dissolved organic carbon (DOC), were taken in 1 L polycarbonate bottles.
Lake sediment cores were collected with a gravity K-B corer and were sliced in the field into 1 cm slices. Each slice was placed in a Whirl-Pak, and frozen within 48 hours.
THg analyses: sediment and crayfish
Frozen samples were thawed, dried, and weighed prior to analyses. THg concentrations were determined using EPA Method 7473, using a DMA-80 mercury analyzer. Reference material used was MESS-3 (Marine sediment, Beaufort Sea, National Research Council, Canada) for THg in sediments and Dorm 3 for crayfish. Reference recoveries are in Table S5.
Cs-137 dating of cores
Sediment Cs-137 was measured by HPGe gamma spectroscopy. Samples were oven dried at 50 °C, ground, compressed at 3000 pounds per square inch into 5 cm diameter cylindrical pellets (5.6—6.9 g dry weight (gdw)), placed in heavy aluminum foil planchets. A series of four similarly prepared National Institute of Standards and Technology (NIST) Cs-137 spiked clay standards with a range of thicknesses and known activities served to calibrate detector efficiencies.
THg and MeHg analyses: water
Samples were analyzed within 48 hours of collection. Filtered samples were filtered through 0.45 μ cellulose nitrate filters, using a Nalgene disposable vacuum filtration system. THg concentrations were analyzed using EPA Method 1631e after BrCl oxidation, SnCl2 reduction, and purge and trap, followed by Cold Vapor Atomic Fluorescence Spectroscopy (CVAFS). MeHg concentrations in water were determined by EPA Method 1630, which involves distillation, ethylation, followed by purge and trap from water samples, gas chromatographic separation of species, and quantification by CVAFS.
THg and MeHg: water quality assurance and control
Field blanks were taken in duplicate at the beginning and end of each sampling day, and in the middle if more than 4 sites were sampled. Field and travel blanks were also done in conjunction with each sampling date. Blank results (Table S1) were all well below the recommended limit of 0.5 ng THg/L (EPA Method 1631). The average relative percent difference (%RPD) for analytical replicates was less than 3%, and the average %RPD for duplicate samples was 6% for unfiltered THg samples (Table S2).
TSS, suspended inorganic particles, and DOC
Water samples were chilled for transport to the lab and filtered within 48 hours, using pre-weighed, combusted Whatman GFF glass microfiber filters (approximate pore size 0.7 μ). For TSS, the residue retained on the filter was dried for 16 hours to a constant weight at 104 ± 3 °C. The increase in the weight of the filter was assumed to be the total suspended solids. The mean detection limit for this analysis is 0.15 mg. For suspended inorganic particles, following TSS measurement, the same filters were combusted at 500 ± 3 °C for 16 hours to a constant weight to remove the organic fraction. The mean detection limit for this analysis is 0.15 mg. For DOC, the filtrate was acidified with H3PO4 to a pH of 2–3. The inorganic carbon component was then sparged with hydrocarbon-free gas and quantified using a Shimadzu TOC-VCPH + TNM-1 Total Organic Carbon and Total Nitrogen Analyzer. The mean detection limit for this analysis is 13 μmol C/L or 0.16 mg C/L.
Chloride and sulfate
Samples were analyzed using a liquid chromatography system (Shimadzu LC- 10AD Liquid Chromatograph). The mean detection limit for chloride is 0.02 mg/L and for sulfate is 0.01 mg/L.
Oxygen and temperature profiles
Oxygen and temperature were measured in the field using a YSI Pro 20i handheld meter.
Estimates of Wabigoon River transport of THg and MeHg from Wabigoon Lake to the Ball Lake outflow
The masses of THg and MeHg transported past each sampling site were calculated as the product of concentration and water flow volumes. These volumes were measured at 3 sites (Fig. 1; Wainwright Dam, Eagle River and at the Water survey of Canada site on the Wabigoon River near Quibell ON (05QD006)), Downriver flows were estimated using Cl− dilution at the remaining water sampling sites, as described in the Supplementary Material.
Crayfish sampling and analyses
Crayfish were collected using baited crayfish traps. After collection they were washed, their length and weight were recorded, and they were then frozen in the field. Concentrations of THg in the abdominal muscle of individuals were determined using the same methods described above for THg in sediments. The percentage of THg that was MeHg was about 88% (Pennuto et al. 2005).
Results
Background concentrations of THg and MeHg in water and sediment
For this study, we define background concentrations for the English–Wabigoon system as those found upstream of the Dryden plant site (Dinorvic and Wabigoon Lakes) and upstream of Ball Lake on the English River (Tide Lake, Fig. 1). In Dinorvic, Wabigoon, and Tide Lakes, surface sediment THg concentrations were 76, 69, and 37 ng/gdw, respectively. Therefore, we define background for sediments as below 80 ng/gdw. Average water concentrations in Wabigoon Lake outflow above the plant site and in the English River inflow to Ball Lake were 1.2 and 1.1 ng THg/L and 0.02 and 0.04 ng MeHg/L, respectively. Therefore, we define background for water as below 1.2 ng THg/L and 0.04 ng MeHg/L.
Longitudinal pattern of THg concentrations in surface sediments of the Wabigoon River
In 2016, mercury concentrations in surface sediments (0–1 cm) were assayed upstream and downstream of the former chlor-alkali plant in Dryden, which is now the site of a paper mill that occupies the riverbank from rkm 1–4.5 (Figs. 1, 2). Mercury concentrations were at background levels (<80 ng/gdw) in Dinorvic Lake and Wabigoon Lake upstream of the plant site. At the plant site there was a sharp increase in surface sediment THg concentration to 13,200 ng/gdw (Fig. 2). This was followed by a steep decline 2 km farther downstream. Much farther downstream in the east basin of Clay Lake surface sediment mercury concentrations were lower (1,300 ng/gdw) but still 16 times above background. This type of longitudinal pattern is consistent with point source contamination suggesting that the plant site might still be an ongoing mercury source to the river, decades after the initial clean-up. However, as shown below, further data collections did not support this.
Fig. 2.

Sources, concentrations, and transport of THg in the English–Wabigoon River water from Wabigoon Lake to Ball Lake outflow.
Wabigoon Lake water, which is upstream of the plant site, was at background THg concentrations (average 1.23 ± 0.2 ng/L; Fig. 3a). The very low upstream unfiltered THg concentrations of Wabigoon Lake water remained at near background levels (1.57 ± 0.39 ng/L) as the river water flowed by the plant site (rkm 3; Fig. 3a). Concentrations had increased by rkm 7.7 and continued to increase downstream as far as Clay Lake (rkm 86), reaching about 10 ng/L soon after ice-off and 15–25 ng/L during summer and fall (Fig. 3a). Unfiltered THg concentrations peaked at the Clay Lake inflow, and then declined on average by 77% to 3.9 ng/L at Clay Lake outflow (Fig. 3a). Concentrations were similar at the inflow to Ball Lake (rkm 147, 4.2 ng/L), but were much lower (1.8 ng/L) at the outflow of Ball Lake (Fig. 3a). This was likely because of mercury sedimentation in Ball Lake and the large dilution from the English River that also flows into Ball Lake. The English River has average background mercury concentrations of 1.1 ng/L, similar to Wabigoon Lake outflow (Table 1, Fig. 1). This general longitudinal pattern occurred whether the river flow rate was high or low (Fig. 3a, Fig. S9a).
Fig. 3.

Table 1.
Volume m3 | THg ng/L | MeHg ng/L | THg loading g | MeHg loading g | |
---|---|---|---|---|---|
Wabigoon River inflow | 1.1 × 109 | 4.2 | 0.20 | 4800 | 220 |
English River inflow | 4.1 × 109 | 1.1 | 0.04 | 4100 | 140 |
Ball Lake outflowa | 5.1 × .109 | 1.8 | 0.09 | 8200 | 400 |
Note
Water volumes were estimated using the chloride dilution method described in the Methods section. THg, total mercury; MeHg, methyl mercury.
a
The outflow of THg and MeHg from Ball Lake is an underestimate because direct inputs to Ball Lake from local drainage were unknown. However, this error is likely small because most of the mercury is transported during times of high river flows.
To further define the pattern of water concentrations directly in front of the plant and just downstream, concentrations in river water were sampled at several additional locations immediately in front of the plant site (Fig. S1). Average concentration of all samples taken from in front of the plant site, including from the mill effluent, was low (1.57 ± 0.39 ng/L, n = 10) compared with the much higher concentrations downstream (Fig. 3a). This average was not statistically different from the THg average concentrations in the outflow of Wabigoon Lake (1.23 ± 0.20 ng/L, n = 6). There may have been some increase in THg concentration at Gordon Road, but the first obvious increase in concentration was between Gordon Road (rkm 4.3) and rkm 5.77 with further increases downstream to Highway 17 (rkm 7.6, Fig. S1). All these water data suggested that the plant site was not, in 2017, an important source of mercury to the river water even though surface sediments in the near vicinity of the mill were still very high in mercury concentration.
In aquatic ecosystems mercury is transported either tightly bound to DOC (Haitzer at al. 2002) or on TSS, especially on very fine suspended particulate matter (Kelly and Rudd 2018). DOC concentrations peaked just below the paper mill, due to high concentrations in the outfall there, and then decreased through dilution, becoming fairly constant by the Clay Lake inflow (Fig. S3c). In contrast, except for the 23–24 July 2017 sampling, TSS increased as the river flowed downstream, then decreased dramatically as particles settled in Clay Lake (Fig. 4, Fig. S3a).
Fig. 4.

In Wabigoon Lake, at background THg concentrations, most of the THg (average of 64%; Figs. 3a–3c) was dissolved and therefore bound to DOC. In contrast, downstream of the plant site most of the THg was on particles, with 80% of the THg entering Clay Lake being on particles. Below Clay Lake, at much lower mercury and TSS concentrations, THg was transported mostly (66%) in the dissolved form.
Overall, the concentrations of both unfiltered THg and particulate THg increased steadily below Dryden. However, as the river flowed through Clay Lake, because of particle trapping, concentrations of unfiltered THg and particulate THg fell on average by 77% and 89%, respectively. The decrease in dissolved THg was much less pronounced (Figs. 3b, 3c).
We also determined the THg content of the particles at the sampling sites (Fig. 3d). In Wabigoon Lake the THg content was very low (averaging 55 ng/g TSS). As the river flowed over the most contaminated sediments at the plant site (Figs. 1, 2) the content of THg in particles increased somewhat to 75 ng/g TSS at rkm 3. Downstream of the plant site at rkm 7.7 content jumped to 390 ng/g TSS and then increased farther downstream, averaging 710 ng/g TSS at Clay Lake inflow. The particulate matter that left Clay Lake and was transported to Ball Lake was both lower in concentration (ng/L, Fig. 3b) and lower in mercury content (ng/g Fig. 3d), indicating that a large portion of the contaminated particles were deposited in Clay Lake (Fig. 3d).
The overall longitudinal patterns for THg concentrations in water were very repeatable on each of the five sampling occasions (Fig. 3).
The mass load of mercury (flow × concentration) that was transported out of Wabigoon Lake and past the plant site was estimated to be 430 g during the ice-free season of 2017 (1 May—30 September, Fig. 5a). The first detectable input to the river occurred at 7.7 km downstream of the mill where the load for the season increased to 2000 g. Thereafter, loading increased steadily to 14 000 g at the inflow to Clay Lake (Fig. 5a).
Fig. 5.

All these data (Figs. 3a, 3d, 5a, and Fig. S1) demonstrate that during our 2017 samplings the plant site was not a significant source of mercury to the upper river. Instead, because of erosion of contaminated floodplain particles, there appeared to be a diffuse source of particulate mercury to the river at a quite consistent net rate of about 160 g/km (Fig. 5a) between rkm 7.7 and the Clay Lake inflow (rkm 86).
Clay Lake was a large sink for THg, of about 10,000 g, because of particle trapping (Figs. 3b, 5a). Most of the mercury that left Clay Lake was transported directly to Ball Lake (Fig. 5a). Ball Lake has two major inflows, the Wabigoon River and the English River (Fig. 1). The Wabigoon River had much smaller inflow volumes, but because of its higher mercury concentrations it was a somewhat larger source of THg to Ball Lake (Table 1).
Below the plant site almost all the measured TSS was suspended inorganic particles (Figs. S2, S3a), which was almost completely retained in Clay Lake (about 90%, Fig. S2). In the English–Wabigoon system, most of the inorganic particles are fine clay-rich material (Armstrong and Hamilton 1973), and it is these very fine clay-particles, that transport mercury efficiently in mercury contaminated ecosystems (Kelly and Rudd 2018). Inorganic particle concentrations averaged about 50% of TSS in Wabigoon Lake and in front of the mill. Below rkm 7.7, suspended inorganic particles increased to 70% of TSS. This suggests that it is inorganic particles (fine clay) that are being remobilized from the contaminated riverbanks and it is inorganic particles that are transporting the THg downstream.
Sources, concentrations, and transport of MeHg down the English–Wabigoon River from Wabigoon Lake to Ball Lake outflow
Unfiltered MeHg concentrations were at background levels (0.02 ng/L) in Wabigoon Lake, and at sampling sites as the river flowed by the plant site (Fig. 6a). Downriver, unfiltered MeHg concentrations began to increase at rkm 7.7. Maximum concentrations were reached at rkm 27, and concentrations remained at these high levels (averaging 0.6 ng/L) until Clay Lake inflow (rkm 86, Fig. 6a). Thereafter, concentrations decreased progressively until Ball Lake outflow where MeHg concentrations were still on average 2.5 times that of the English River inflow (Fig. 6a, Table 1).
Fig. 6.

A major difference between THg and MeHg in the river water was that, in the most contaminated reaches of the river between Dryden and Clay Lake, 85% of the MeHg was in the dissolved form (Figs. 6a –6c), unlike THg that was 80% on particles (Figs. 3a–3c).
We estimated sources, sinks, and mass transport of MeHg down the river using the same approaches as for THg. Most of the MeHg being transported in the river system was produced between Dryden and Clay Lake (Fig. 5b). Because most of this MeHg was soluble, a large proportion of the MeHg produced between Dryden and Clay Lake was transported through Clay Lake and downstream towards Ball Lake (Fig. 5b), near where the Grassy Narrows community lives and, in the past, did most of their subsistence fishing and guiding.
The load of MeHg entering Ball Lake was twice as high from the Wabigoon River as from the English River, even though the flow volume was much lower (Table 1).
Mercury concentrations in crayfish
The impact of the elevated mercury in the inflow from the Wabigoon River to the south basin of Ball Lake (Figs. 3, 6) was evident in crayfish sampling from Ball Lake (Fig. S10). THg concentrations in abdominal muscle samples (approx. 88% MeHg) were low in Tide Lake, which is upstream of Ball Lake on the English River, and in the north basin of Ball Lake, which is heavily influenced by the lower mercury concentrations in the inflow of the English River to Ball Lake. At the Wabigoon River inflow to Ball Lake and at the Ball Lake outflow, crayfish mercury concentrations were more than twice as high as at the other sampling sites (Fig. S10). MeHg in water was also more elevated at these two sites (Table 1).
Hypolimnetic MeHg accumulation in the west basin of Clay Lake
The west basin of Clay Lake stratifies, and in 2017 oxygen was progressively depleted in its bottom waters during the summer and fall. The water was anoxic below 15 m by late September (Fig. 7a). During August, MeHg began to accumulate below 13 m and concentrations reached 5.3 ng/L at 1 m above the sediment–water interface (19 m) by late September. In late September, as the thermocline descended (Figs. 7a, 7b), the accumulated MeHg in the deep waters of the west basin was mixed with surface water, increasing the MeHg concentrations (Figs. 6a, S8b) and the concentration of MeHg in particles at the outflow (Figs. 6a, 6d). A significant portion of this MeHg was likely transported downstream to Ball Lake.
Fig. 7.

Ball Lake remained unstratified and well oxygenated all summer and therefore there was no hypolimnetic MeHg accumulation in the water column.
Deposition in Clay Lake and Ball Lake sediments
We, and several other researchers, have followed the deposition of THg at the same central location in the east basin of Clay Lake since 1971 (Fig. 8a, Fig. S4). In 1971, which was one year after the chlor-alkali plant discharge was regulated, the highest mercury concentration (8400 ng/gdw) was in the surface sediments. By 1980 the peak in mercury concentrations had been buried to a depth of 3.5 cm (Fig. 8a). Successive long cores in 1995 and 2004 found that the peak had been buried to 8 cm and 9.5 cm, respectively. We cored the same site again in 2017 and the peak mercury concentration was found at 18.5 cm below the sediment–water interface (Fig. 8a). The increased rate of burial between 2004 and 2017 suggests that there has been an increased rate of sediment accumulation at this site since 2004.
Fig. 8.

The 2017 core was assayed for Cs-137 content (Fig. S4) as was the 1995 core (Lockhart et al. 2000). Peaks in Cs-137 concentration, which indicate sediments deposited in 1963, were found just below the peaks in mercury concentration in both cores. The 2017 data confirm the conclusion of Lockhart et al. (2000) that mercury does not migrate vertically in Clay Lake sediments and that the peak mercury concentrations occurred in the late 1960s in the same time frame as the closure of the chlor-alkali plant in Dryden (1969)—followed by progressive burial until the present, as the site slowly recovers.
Shorter cores were taken, in 2017, at four additional sites in the east basin and 5 sites in the west basin of Clay Lake (Fig. S5). Surface sediment concentrations in the east basin were more than twice those of the west basin (averaging 1,200 and 520 ng/gdw, respectively, Fig. S6), which is consistent with higher mercury content particles sedimenting soon after their arrival in Clay Lake from the Wabigoon River (Fig. 3d). All cores had a continuous decrease in mercury concentrations towards the surface (Fig. S6), indicating an ongoing recovery from mercury pollution in sediment throughout Clay Lake, but these concentrations are still 15 and 7 times higher than background concentrations.
The historical surface sediment concentrations of THg at the central location in the Clay Lake east basin show that there was a rapid recovery of surface sediment concentrations between 1971 and the 1980s (Fig. 8b). Thereafter, the system has recovered at a much slower rate. Walleye concentrations in muscle decreased quickly until the early 1980s but have plateaued at about 2,000 ng/g wet weight since then (Fig. 8b).
In contrast to Clay Lake where sediment mercury concentrations are decreasing, cores taken in 2007 from the south basin of Ball Lake had increasing concentrations of mercury towards the surface (Fig. 9). This indicates that at that time pollution of Ball Lake was still increasing as the mercury was transported downstream from Clay Lake where sediment mercury concentrations were higher. Similar delays to downstream transport of mercury from chlor-alkali point sources, i.e., decreasing in sites near the original contamination while increasing in more distant sites, have been observed in other river systems (Santschi et al. 2017).
Fig. 9.

Sediment THg concentrations were uniform with depth and at background levels in the north basin of Ball Lake and in Tide Lake, which is upstream of Ball Lake (Fig. 9). These areas receive inflow from the English River (which has a background mercury concentration of 1.1 ng/L, Table 1), and are upstream of influence from the Wabigoon River.
Discussion
Present-day sources of mercury to the English–Wabigoon River system
Fifty-seven years after mercury discharges to the river began in 1963 at Dryden, the English–Wabigoon River system remains polluted by mercury. Forty years after the last comprehensive sampling of the English–Wabigoon system, concentrations of mercury in Clay Lake sediments and inflow water have declined but are still 15 times above background concentrations (Figs. 3, 8a, 8b), and wet weight mercury concentrations in fish are at levels found only in contaminated sites (DePew et al. 2013). This pollution is also evident even farther downstream (150 km from the plant site), where MeHg concentrations in the Ball Lake inflow from the Wabigoon River and outflow are 10 times and 2.5 times above background concentrations respectively (Fig. 6a).
Our water data demonstrate that at time of our sampling the ongoing contamination of the river water was not caused by leakage from the current plant site, but instead was caused by erosion of mercury contaminated clay particles from the riverbanks and floodplain in a reach of the river beginning about 5 rkm downstream of the plant site and continuing until Clay Lake inflow. This conclusion became evident when we saw no increase in mercury load as the river flowed by the plant site. In contrast, there were progressive large increases in loads of both THg and MeHg beginning downstream of rkm 5 and continuing as the river flowed towards Clay Lake (Figs. 5a, 5b; Fig. S1). In the case of THg, the most reasonable explanation for the fact that there was both an increase in the load of THg (Fig 5a), and an increase in the average THg content of the suspended particles (Fig. 3d), is that there was an addition of particles that were much more contaminated than the clean particles entering the river from Wabigoon Lake.
A model predicted that the added particles were on average 1,200 ng THg/g (Fig. 10), which is in the range of measurements of floodplain soils (Table S4). These particles were most likely clay (Armstrong and Hamilton 1973), and our data showed that most of the increase in TSS as the river flowed downstream was due to suspended inorganic particles (Figs. S2 and S3a).
Fig. 10.

Periodic flooding during the past 5 decades has redistributed the legacy THg discharged from the plant between 1962 and 1969, contaminating the riverbanks and floodplains. The flood plains (Fig. S7) are located behind the riverbanks, and soil samples showed high THg concentrations (Table S4). While the exact extent of this contamination is unknown, our loading data suggest that it is the most probable source of the continued THg input to the river. This is because the Wabigoon River forms meanders over time because of cut-bank erosion. This process brings new contaminated particles into the river, increasing the load of both TSS and THg as it flows towards Clay Lake.
The longitudinal pattern of a point source input looks very different from the THg and TSS patterns, described above. For example, DOC and Cl− (Figs. S3b, S3c) are known point sources from the treatment pond of the currently operating mill. Both constituents showed peaks in concentration at the first sampling site below the plant site, because they are both present in high concentration in the treatment pond effluent (rkm 3.5). As the river flows downstream and becomes diluted with natural runoff, these point source concentrations decrease.
The longitudinal pattern of mercury in river water was also different than the longitudinal pattern in sediments (Fig. 2). The highly elevated mercury concentrations in surface sediments in front of the plant site may be the result of very slow ground water discharges from the decommissioned chlor-alkali plant site to the river, or they may simply be the remainder of mercury discharged in the 1960s. No matter the cause, a thorough cleanup of the site is needed because it remains a large potential source of mercury to the river. A very similar situation was seen in the Penobscot Estuary in Maine, where there were very high mercury concentrations in sediments in front of a previous chlor-alkali plant, but the ongoing contamination of the estuary was being caused by widespread erosion and redistribution of downstream legacy mercury (i.e., mercury that had been discharged while the chlor-alkali plant was operating; Rudd et al. 2018). Large deposits of fugitive mercury were found under the concrete pads of the chlor-alkali cells at the Penobscot site, but these contaminated soils have since undergone extensive site remediation. We recommend that removal of the concrete pad and remediation of the underlying soils be done at the Dryden plant site.
It is possible that there are intermittent mercury discharges and (or) erosion of high mercury sediments in front of the plant site (Fig. 2) at times when we were not sampling the river, which could be an additional mercury source to the river. This possibility could be investigated with short-time interval sampling over long time periods, just downstream of the plant site.
MeHg production in the English–Wabigoon system
THg is converted to MeHg in anoxic habitats by different types of micro-organisms (iron reducers, sulfate reducers, and methanogens; Bravo et al. 2018). In contaminated ecosystems, such as the English–Wabigoon, the production of MeHg is positively related to the concentration of THg in sediments (Rudd et al. 2018). Because MeHg is so toxic to humans and wildlife, it is important to understand where it is produced in ecosystems, so that its production can be minimized.
We hypothesized before this study that much of the MeHg was being produced in the extensive wetland areas that border the upper river between Dryden and Clay Lake between rkm 46 and rkm 61 (Fig. S7). We hypothesized this because the low-lying nature of wetlands means that they must have been flooded periodically during the years of high mercury contamination from the chlor-alkali plant, and because many wetlands in northwestern Ontario are important sources of MeHg to downstream ecosystems (St. Louis et al. 1994; St. Louis et al. 1996). However, the data reported here do not support this hypothesis. Most of the MeHg production must have occurred in the river channel (i.e., the sediments and (or) banks) because the rate of increase in load of MeHg in the river, per km, was constant between Dryden and Clay Lake (Fig. 5b) whether the river was flowing through woodlands and farmlands or through the wetland areas. This conclusion is also supported by our sampling of 6 tributary streams that drained the wetlands during August, when methylation rates peak in NW Ontario wetlands (St. Louis et al. 1994). With one exception, MeHg concentrations were lower in the tributary streams than in the mainstem of the river (Table S3), supporting our conclusion that the wetlands were not sources of MeHg to the river.
For remediation purposes, it’s important to understand exactly where MeHg is being produced in the river channel between Dryden and Clay Lake. We think that methylation of legacy mercury was most likely occurring in sediment floc that accumulates on the bed of the river in quiescent areas and possibly in piles of wood debris, where currents are slow. Sediment flocs are highly microbially active (Sweerts et al. 1986) and therefore become anoxic soon after deposition. MeHg production ensues and the newly formed MeHg accumulates temporarily until the river current changes and the floc along with recently produced MeHg is swept into the overlying river water. This hypothesis is supported by work in the East Fork of the Poplar Creek—a mercury contaminated river in Tennessee. There, stable isotopic studies found that legacy inorganic mercury found in recalcitrant forms in the creek banks was also found in more soluble forms in the creek bed that would be more available for methylation (Crowther et al. 2021). Methylation has also been observed in riverbank soils (Abdelmageed et al. 2021) and in wood debris (Kelly and Rudd 2018).
The lack of a large effect of the wetlands on MeHg concentrations in the river water does not mean that there is not active methylation in these wetlands. Instead, it could be that these clay-rich wetlands are operating in a manner similar to the clay-rich wetlands in the Penobscot Estuary where MeHg is actively produced (Gilmour et al. 2018), but is retained in the wetlands (Turner et al. 2018), where it impacts birds and other wildlife living in the wetlands (Kopec et al. 2018; Sullivan and Kopec 2018). Therefore, these wetlands should be studied and remediated, if necessary, to ensure that resident wildlife are not at risk.
While the river between Dryden and Clay Lake was the most important site of MeHg production in the system (Fig. 5b), another important site was the hypolimnion of the west basin of Clay Lake, which is anoxic at its lower depths during late summer and fall (Fig. 7a); 95 g of MeHg had accumulated in the bottom waters before fall overturn (Fig. 7b). However, Clay Lake was a net sink for MeHg; 430 g entered the lake from upstream, but only 210 g exited during our May to September sampling period (the difference being MeHg deposition and degradation). So, while 95 g accumulated in the hypolimnetic water it is likely that this accumulation was from a combination of MeHg production and deposition from the epilimnion. Whatever the origin, however, it is mostly dissolved and would be swept into the epilimnion during fall circulation and contribute to the outflow of MeHg, as we saw in our last sampling of the outflow in September (Fig. S8b).
The finding that Clay Lake was a sink rather than a source of MeHg to the river does not mean that there is no MeHg production in the epilimnetic sediments of Clay Lake. Epilimnetic sediments are important sites of MeHg production (Ramlal et al. 1993), and it is available to the benthic infauna (e. g. mayflies) in subsurface sediments. These infauna are consumed by fish after their emergence.
Overall, we conclude that throughout the spring and summer stratification, most of the MeHg was produced between Dryden and Clay Lake, and because it was dissolved, it was transported easily throughout the system as far as Ball Lake. Thus, this upstream reach should be a primary focus for remediation of MeHg production in the system.
Ball Lake sediments were not a significant source of MeHg to the water (Table 1), but similar to Clay Lake there must have been MeHg production in the sediments, which had elevated THg concentrations in the south basin (Fig. 9). This likely impacted the benthic food web and thus was a source of MeHg to the fish in Ball Lake. Remediation of this situation is discussed below.
Similarities and differences between the English–Wabigoon of the late 1970s and 2017
During the 40 years since the English–Wabigoon system was last intensively sampled, concentrations of THg in water and sediment have decreased by about 50% (Fig. 8b). For example, during summer at Clay Lake inflow, THg concentrations averaged 30 ng/L in 1978–1980 (Parks et al. 1986), and had decreased to 15 ng/L in 2017 (Fig. 3a). MeHg concentrations had decreased by about a factor of 4 (2.5 to 0.6 ng/L, Fig. 6a, Parks et al. 1989). But these present-day concentrations at Clay Lake inflow are still very high—both about 15-fold higher than background concentrations seen in Wabigoon Lake and the English River as it enters Ball Lake (Figs. 3a, 6a, Table 1).
Although concentrations are lower than in the late 1970s, the patterns of longitudinal and seasonal mercury behavior in the system itself are remarkably similar to the late 1970. For example, there was some ongoing loss of mercury from the effluent of the plant in the late 1970s, but it was concluded, as we do, that this loss was small in comparison to erosion of mercury from downstream floodplain and riverbank soils. Both studies found a large build-up of THg and MeHg in the river water between Dryden and Clay Lake, and that much of the THg, but not MeHg, was trapped in Clay Lake. This was because THg was mostly particle bound between Dryden and Clay Lake, whereas MeHg was mostly dissolved throughout the system.
In both studies, MeHg production was intense in the river between Dryden and Clay Lake and the resulting high concentrations in river water impacted concentrations in the eastern basin of Clay Lake and in downstream reaches as far as Ball Lake. Also, in both studies, there was active MeHg accumulation in the hypolimnion of the west basin of Clay Lake and this increased MeHg concentrations and transport to Ball Lake after overturn. As is now the case, THg concentrations at Clay Lake outflow and Ball Lake inflow from the Wabigoon River (47 km farther downstream) were similar.
THg and MeHg concentrations in the late 1970s were very seasonal, with low concentrations in the winter and spring, and with much higher concentrations during summer and fall months (Parks et al. 1986). Similarly, in 2017, THg and MeHg concentrations at ice-off were low in the upper river and were much higher during the summer and fall (Figs. 3a, 6a). The seasonality of MeHg production is to be expected (Ramlal et al. 1993; Kelly et al. 1997), but the reasons for seasonal changes in THg concentrations in both studies are unclear.
The similarities over time, and therefore predictability, of mercury transport and methylation in the English–Wabigoon system should facilitate the construction of conceptual and mechanistic models of mercury transport and methylation in the ecosystem, and these models will facilitate remediation (our conceptual model of THg and MeHg in the English–Wabigoon system can be found in Supplementary Material).
Remediation
The 1980’s federal–provincial study recommended remediation using the science-based approaches developed during that study (Rudd et al. 1983; Rudd and Turner 1983). Instead, the government opted to wait for natural recovery. Some natural recovery in the sediments has occurred during the intervening decades, with the rate being rapid at first and then slowing to a very low rate. Fish also recovered rapidly at first but have not decreased since the mid 1980s (Fig. 8b, Neff et al. 2012). The divergence of fish recovery from sediment recovery is likely linked to the continuing inputs of MeHg to Clay Lake from upstream. Other factors may also be at play, such as the extensive logging of the watershed of Clay Lake (Fig. S5, Wu et al. 2018).
The current rate of recovery is much too slow both in an ecological sense and for the Grassy Narrows people. Their commercial fishery remains closed and their health and livelihood continues to be impacted (Mosa and Duffin 2017; Harada et al. 2011; Philbert et al. 2020). Therefore, active remediation of the English–Wabigoon system is still necessary.
The erosion of mercury from riverbanks between Dryden and Clay Lake, and its downstream transport, elevates surface sediment inorganic mercury concentrations throughout the system. This elevation stimulates MeHg production (Rudd et al. 2018).
The goal of remediation is to reduce the sources of MeHg to fish. In this system, there are three MeHg sources: MeHg produced between Dryden and Clay Lake, which is then swept down the river as far as Ball Lake; hypolimnetic production in Clay Lake; and production in the epilimnetic sediments of Clay Lake and Ball Lake. Our mass-transport MeHg data (Fig. 5b) suggest that most of the MeHg production in the system occurs between Dryden and Clay Lake, which elevates MeHg concentrations in river water throughout the system (Fig. 6a). This upstream source is the most important and so should be a primary focus of remediation. Secondary remediations for Clay Lake and Ball Lake are also discussed below.
Possible remediation measures for the English–Wabigoon system were reviewed recently (Rudd et al. 2016), and the more promising approaches were recommended to the Ontario government (Rudd et al. 2018). Extensive dredging was not recommended because of ecological risk. The mercury buried in Clay Lake sediments has not moved in five decades and is unlikely to in the future (Fig. 8a). Armouring of riverbanks to prevent erosion between Dryden and Clay Lake would disrupt the extensive wetlands by changing the hydrology that they depend upon. Also, it seems impractical because our data suggest that the mercury contamination is widespread in the floodplains—there are 180 km of riverbanks between Dryden and Clay Lake inflow that would need to be armoured. These large-scale types of engineering activities have caused reversals in ecosystem recovery (NRC 2007).
One area that could be explored is reducing the high concentrations of DOC or sulfate in the plant effluents (Fig. S3 c, d). However, these remediation possibilities are not straightforward solutions. It is possible that the DOC in plant effluents is solubilizing inorganic mercury in contaminated bank sediments thereby making it more available for methylation, but elevated DOC concentrations have also been shown to inhibit MeHg production because some kinds of DOC bind inorganic mercury (Hg2+) rendering it less available for the mercury methylators (Miskimmin et al. 1992). Similarly, lowering sulfate in plant effluents is not straightforward. Lowering sulfate concentrations might reduce mercury methylation because sulfate reducing bacteria are known to methylate mercury, and MeHg production was stimulated at the Experimental Lakes Area (ELA) by sulfate additions (Branfireun et al. 2005). However, additions of sulfate to enclosures in Clay Lake lowered MeHg production rates presumably because of binding of mercury by sulfide (Rudd et al. 1980). Because of this conflicting information, if more tested approaches are available, it seems that these should be tried first.
Two approaches that have already been demonstrated for remediation of mercury contamination in ecosystems are: (i) hypolimnetic additions of nitrate and (ii) enhanced natural recovery (ENR) by addition of clean (low mercury) lacustrine clay to the upper river.
Hypolimnetic additions of nitrate have been shown to reduce production of MeHg in lakes (Matthews et al. 2013), because the presence of nitrate increases the redox potential of the surface sediments where methylation occurs. Nitrate additions to Clay Lake would lower the accumulation of MeHg in the bottom waters of Clay Lake, which would reduce the MeHg available for bioaccumulation from water and particles by the food chain and fish after fall circulation (Paterson et al. 1998). This would also reduce the amount of MeHg that would be transported downstream to Ball Lake by about 50%.
Alternatively, hypolimnetic oxygen additions, which have long been used to control eutrophication, would also increase redox potential, but this approach has not yet been tried for control of MeHg production.
ENR is the addition of clean lacustrine clay to an ecosystem to reduce MeHg production (Rudd et al. 1983, 2018). Additions of clean lacustrine clay from Wabigoon Lake to 10 m diameter enclosures in Clay Lake reduced the production and bioaccumulation of MeHg by 90% (Rudd and Turner 1983). This approach reduced MeHg production in two ways. First, the dilution of highly contaminated sediments with clean clays decreased the concentration of THg. Second, it was found that the fine clay particles, which were taken from the surface sediments of Wabigoon Lake, were highly efficient at binding added inorganic THg, possibly because of coatings of organic molecules on the surfaces of the clay. Thus, the decrease in methylation was more than expected simply due to the dilution of THg.
Because of the ongoing erosion of contaminated particles from the floodplains between Dryden and Clay Lake, neither of these approaches would work as single applications. Production of MeHg could be reduced in the system by a once-a-year addition of nitrate to the Clay Lake hypolimnion and by continuous small-scale resuspension of clean sediments (ENR) from Wabigoon Lake into the river at Dryden.
In the case of ENR, clean mercury-binding particles would be redistributed naturally from the outflow of Wabigoon Lake to downstream areas, including the upper river where they would be deposited to the same quiet areas where microbially active floc accumulates (as described above). They would also be deposited onto the surface sediments of Clay Lake. Additions could be done during summer when methylation rates are at their highest.
The river between Dryden and Clay Lake is highly controlled by dams at the outflows of Wabigoon and Eagle Lakes (Fig. 1). Erosion rates, which add particulate mercury to the river, are often positively related to current velocity, and indeed at times in the Wabigoon River there were higher concentrations of particles and mercury at higher flow rates (Fig. S12). If peak flow rates (Fig. S9) were reduced, this would decrease the supply of legacy mercury to the river, which would decrease THg concentrations in the river and in Clay Lake. This in turn would lower rates of MeHg production. Controlling river flow would also reduce downstream flooding that simulates MeHg production (St. Louis et al. 2004).
Remediation in Ball Lake is also necessary. The north basin of Ball Lake is quite clean because it receives large volumes of clean water from the English River (Figs. 1, 9, Table 1). Sediment and biota concentrations (Fig. 9, Fig. S10) are much higher in the south basin that receives elevated concentrations of THg and MeHg from the Wabigoon River inflow to Ball Lake (Figs. 1, 3, 6). The south basin is an important traditional subsistence fishing location for the Grassy Narrows community.
There are two options for remediating the south basin of Ball Lake. First, concentrations of MeHg and THg in the Wabigoon River inflow to the south basin of Ball Lake are presently high (Figs. 3, 6), but they would both be lowered if the previously described upstream remediations for the river and Clay Lake were carried out. Second, ENR could also be applied to the south basin by resuspending clean clay-rich sediments from the north basin, where mercury concentrations in sediments are very low (Fig. 9).
The ultimate long-term remediation goal should be to reduce fish mercury concentrations to levels that will enable the Grassy Narrows people to resume their subsistence fishery (i.e., 0.2 ppm, wet weight). The best chance of achieving this goal would be to use a combination of approaches including ENR (between Dryden and Clay Lake and in Ball Lake), hypolimnetic additions of oxidants (nitrate or oxygen), and possibly controlling peak flows in the upper river to reduce erosion of contaminated riverbanks and flooding. These science-based approaches, if done annually, would decrease MeHg exposure to biota in the long term. Further, these approaches are benign, and would cause a minimum of disruption to the ecosystem, as opposed to large scale dredging or bank armoring. In addition to these whole ecosystem approaches, the plant site where high concentrations of mercury persist should also be remediated.
Acknowledgments
We thank the Asubpeeschoseewagong Netum Anishinabek (Grassy Narrows) First Nation for their advice and support throughout this project. In particular, we thank the Grassy Narrows River Team, headed by Judy DaSilva, for their help in the design of the river sampling, knowledge of the river system, and assistance in sampling sediments and water from the river. Additional members of the River Team were Shoon Keewatin, Brenda Kokopenace, Fred Land, Harry Fobister, and Bridgette Fobister, and youth members were Kendra Sneaky, Darwin Fobister, Clyde Meeseewaypetung, and Nicole Keesick. D. W. Schindler, D. Suzuki, R. Hesslein, A.L. Hamilton, K. Yeager, D. Sone and A. Telford generously provided their ideas, constructive comments and encouragement on many occasions. R. Harris was an important contributor to both experimental design and data interpretation. Pamela Sellers, Mikayla Sellers-Wiebe, Chris Hauser, and Ben Ratuski of River Air provided dedicated field support and unflagging encouragement. We benefitted greatly from the expert and timely analyses of mercury samples by the staff of Flett Research Ltd. (Dawn Gilbert, Zorica Bilandzija, Jason Stetski, and Xiang Wei). The expert advice and analyses of water chemistry samples by the Freshwater Institute Chemistry Laboratory (Patricia Ramlal, Michael Stainton, and Paul Marten) is also very much appreciated. S. Rudd assisted in coordinating ideas, and M. Parker provided graphic expertise.
This research was funded by Grassy Narrows Chief and Council using a Transfer Payment (TSRF1618-02) provided by the Government of Ontario.
References
Abdelmageed Y, Miller C, Sanders C, Egbo T, Johs A, and Robertson B. 2021 Assessing microbial communities related to mercury transformations in contaminated streambank soils. Water, Air and Soil Pollution, 232: 31.
Armstrong FAJ, and Hamilton AL. 1973. Pathways of mercury in a polluted northwestern Ontario lake. In Trace Metals and Metalloorganic Interactions in Natural Waters. Ann Arbor Science Publishers. pp. 131–157.
Branfireun BA, Krabbenhoft DP, Hintelmann H, Hunt RJ, Hurley JP, and Rudd JWM. 2005. Speciation and transport of newly deposited mercury in a boreal forest wetland: A stable mercury isotope approach. Water Resources Research, 41: W06016.
Bravo AG, Peura S, Buck M, Ahmed O, Mateos-Rivera A, Ortega SH, et al. 2018. Methanogens and Iron-Reducing Bacteria: the Overlooked Members of Mercury-Methylating Microbial Communities in Boreal Lakes. Applied and Environmental Microbiology, 84: e01774–18.
Crowther ER, Demers JD, Blum JD, Brooks SC, and Johnson MW. 2021. Use of sequential extraction and mercury stable isotope analysis to assess remobilization of sediment-bound legacy mercury. Environment Science Processes and Impacts, 23.
Depew DC, Burgess NM, Anderson MR, Baker R, Bhavsar S, Bodaly RA, et al. 2013. An overview of mercury concentrations in freshwater fish species: A national fish mercury dataset for Canada. Canadian Journal of Fisheries and Aquatic Sciences, 70: 436–451.
Fisheries and Oceans Canada, Contaminants in Fish Data Base. 2021. [online]: Available from open.canada.ca/data/en/dataset/18f71c8f-09a3-4d91-bd74-481d17260492.
Gilmour C, Bell T, Soren A, Riedel G, Riedel G, and Calhoun AD, et al. 2018. Distribution and biogeochemical controls on net methylmercury production in Penobscot River Marshes and Sediments. Science of the Total Environment, 640–641: 555–569.
Harada M, Hanada M, Tajiri M, Inoue Y, Hotta M, Fujino T, Takaoka S, et al. 2011. Mercury Poisoning in First Nations Groups in Ontario, Canada 35 years of Minamata Disease in Canada. Journal of Minamata Studies, 3: 3–30.
Haitzer M, Aiken GR, and Ryan JR. 2002. Binding of Mercury (II) to Dissolved Organic Matteer: The role of the mercury-to-DOM concentration ratio. Environmental Science and Technology, 36: 3564–3570.
Hutchison G, and Wallace D. 1977. Grassy Narrows. Van Nostrand Reinhold Lt. 178 pp.
Kelly CA, Rudd JWM, Bodaly RA, Roulet NR, St VL, and Louis A. 1997. Increases in fluxes of greenhouse gases and methyl mercury following flooding of an experimental reservoir. Environmental Science and Technology, 31: 1334–1344.
Kelly CA, and Rudd JWM. 2018. Transport of mercury on the finest particles results in high sediment concentrations in the absence of significant ongoing sources. Science of The Total Environment, 642: 1471–1479.
Kinghorn A, Solomon P, and Chan HM. 2007. Temporal and spatial trends of mercury in fish collected in the English–Wabigoon river system in Ontario, Canada. Science of The Total Environment, 372: 615–623.
Kopec AD, Bodaly RA, Lane OP, Evers DC, Leppold AJ, and Mittelhauser GH. 2018. Elevated mercury in blood and feathers of breeding marsh birds along the contaminated lower Penobscot River, Maine, USA. Science of the Total Environment, 634: 1563–1579.
Lockhart WL, Macdonald RW, Outridge PM, Wilkinson P, DeLaronde JB, and Rudd JWM. 2000. Tests of the fidelity of lake sediment core records of mercury deposition to known histories of mercury contamination. Science of The Total Environment, 260: 171–180.
Matthews DA, Babcock DB, Nolan JG, Prestigiacomo AR, Effler SW, Driscoll CT, et al. 2013. Whole-lake nitrate addition for control of methylmercury in mercury-contaminated Onondaga Lake, NY. Environmental Research, 125: 52–60.
Miskimmin BM, Rudd JWM, and Kelly CA. 1992. The influence of dissolved organic carbon, pH and respiration on mercury methylation and demethylation in lake water. Canadian Journal of Fisheries and Aquatic Sciences, 49: 17–22.
Mergler D, Anderson HA, Chan LHM, Mahaffey KR, Murray M, Sakamoto M, et al. 2007. Methylmercury exposure and health effects in humans: A worldwide concern. Ambio, 36: 3–11.
Mosa A, and Duffin J. 2017. The interwoven history of mercury poisoning in Ontario and Japan. Canadian Medical Association Journal, 189: E213–E21.
National Research Council. 2007. Committee on sediment dredging at superfund megasites, sediment dredging at superfund megasites: Assessing the effectiveness. [online]: Available from nap.edu/catalog/11968.html. 316 p.
Neff MR, Bhavsar SP, Arhonditsis GB, Fletcher R, and Jackson DA. 2012. Long-term changes in fish mercury levels in the historically impacted English-Wabigoon River system (Canada). Journal of Environmental Monitoring, 14: 2327–2337.
Parks JW, and Hamilton AL. 1987. Accelerating recovery of the mercury-contaminated Wabigoon/ English System. Hydrobiologia, 149: 159–188.
Parks JW, Sutton JA, and Lutz A. 1986. Effect of point and diffuse source loadings on mercury concentrations in the Wabigoon River: evidence of a seasonally varying sediment-water partition. Canadian Journal of Fisheries and Aquatic Sciences, 43: 1426–1444.
Parks JW, Lutz A, and Sutton JA. 1989. Water column methylmercury in the Wabigoon/English River-Lake system: factors controlling concentrations, speciation and net production. Canadian Journal of Fisheries and Aquatic Sciences, 46: 2184–2202.
Paterson MJ, Rudd JWM, and St Louis V. 1998. Increases in Total and Methylmercury in Zooplankton following Flooding of a Peatland Reservoir. Environmental Science and Technology, 32, 3868–3874.
Pennuto CM, Lane OP, Evers DC, Taylor RJ, and Loukmas J. 2005. Mercury in the Northern Crayfish, Orconectes virilis (Hagen), in New England, USA. Ecotoxicology, 14: 149–162.
Philbert A, Fillion M, and Mergler D. 2020. Mercury exposure and premature mortality in the Grassy Narrows First Nation community: A retrospective longitudinal study. The Lancet Planetary Health, 4: e141–e148.
Ramlal PS, Kelly CA, Rudd JWM, and Furutani A. 1993. Sites of methyl mercury production in remote Canadian Shield lakes. Canadian Journal of Fisheries and Aquatic Sciences, 50: 972–979.
Rudd JWM, Turner MA, Furutani A, Swick AL, and Townsend BE. 1980. Studies on mercury remediation of the English-Wabigoon River. In: Mercury Pollution in the Wabigoon-English River System of Northwestern Ontario and Possible Remedial Measures. A Progress Report for 1978-79, Prepared by the Steering Committee.
Rudd JWM, and Turner MA. 1983. The English–Wabigoon River system: II. Suppression of mercury and selenium bioaccumulation by suspended and bottom sediments. Canadian Journal of Fisheries and Aquatic Sciences, 40: 2218–2227.
Rudd JWM, Turner MA, Furutani A, Swick AL, and Townsend BE. 1983. The English–Wabigoon River system: I. A synthesis of recent research with a view towards mercury amelioration. Canadian Journal of Fisheries and Aquatic Sciences, 40: 2206–2217.
Rudd JWM, Harris R, and Sellers P. 2016. Wabigoon - English River System Advice on Mercury Remediation - Final Report. Report submitted to: Asubpeeschoseewagong Netum Anishinabek (Grassy Narrows First Nation) – Ontario – Canada Working Group on concerns Related to Mercury.
Rudd JWM, Harris R, Kelly CA, Sellers P, and Townsend B. 2017. Proposal to clean-up (remediate) mercury pollution in the English-Wabigoon River. Prepared for the Asubpeeschoseewagong Netum Anishinabek (Grassy Narrows First Nation) and the Government of Ontario.
Rudd JWM, Bodaly RA, Fisher NS, Kelly CA, Kopec D, and Whipple C. 2018. Fifty years after its discharge, methylation of legacy mercury trapped in the Penobscot Estuary sustains high mercury in biota. Science of the Total Environment, 642: 1340–1352.
Santschi PH, Yeager KM, Schwehr KA, and Schindler KJ. 2017. Estimates of recovery of the Penobscot River and estuarine system from mercury contamination in the 1960’s. Science of the Total Environment, 596–597: 351–359.
Sharpe DR, Pullen SE, and Warman TA. 1992. A basin analysis of the Wabigoon area of Lake Agassiz, a quaternary clay basin in northwestern Ontario. Geographie Physique et Quaternaire, 46: 295–309.
St. Louis VL, Rudd JWM, Kelly CA, Beaty KG, Bloom NS, and Flett RJ. 1994. The importance of wetlands as sources of methyl mercury to boreal forest ecosystems. Canadian Journal of Fisheries and Aquatic Sciences, 51: 1065–1076.
St. Louis VL, Rudd JWM, Kelly CA, Beaty KG, Bloom NS, Flett RJ, and Roulet NT. 1996. Production and loss of methylmercury and loss of total mercury from boreal forest catchments containing different types of wetlands. Environmental Science and Technology, 30: 2719–2729.
St. Louis VL, Rudd JWM, Kelly CA, Bodaly RA, Paterson MJ, Beaty KG, et al. 2004. The rise and fall of mercury methylation in an experimental reservoir. Environmental Science and Technology, 38: 1348–1358.
Sweerts JP, Rudd JWM, and Kelly CA. 1986. Metabolic activities in flocculent surface sediments and underlying sandy littoral sediments. Limnology and Oceanography, 31: 330–338.
Sullivan KM, and Kopec AD. 2018. Mercury in wintering American black ducks (Anas rubripes) downstream from a point-source on the lower Penobscot River, Maine, USA. Science of The Total Environment, 612: 1187–1199.
Turner RR, Mitchell CPJ, Kopec AD, and Bodaly RA. 2018. Tidal fluxes of mercury and methylmercury for Mendall Marsh, Penobscot River estuary, Maine. Science of The Total Environment, 637–638: 145–154.
Vecsey C.1987. Grassy Narrows Reserve: Mercury pollution, social disruption, and natural resources: a question of autonomy. American Indian Quarterly, 11: 287–314.
Wu P, Bishop K, von Brömssen C, Eklöf K, Futter M, Hultberg H, et al. 2018. Does forest harvest increase the mercury concentrations in fish? Evidence from Swedish lakes. Science of The Total Environment, 622–623: 1353–1362.
Supplementary material
Supplementary Material 1 (DOCX / 2.58 MB)
- Download
- 2.58 MB
Information & Authors
Information
Published In
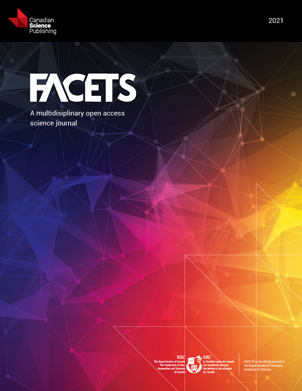
FACETS
Volume 6 • Number 1 • January 2021
Pages: 2002 - 2027
Editor: Clément pierre Bataille
History
Received: 1 July 2021
Accepted: 7 October 2021
Version of record online: 9 December 2021
Copyright
© 2021 Rudd et al. This work is licensed under a Creative Commons Attribution 4.0 International License (CC BY 4.0), which permits unrestricted use, distribution, and reproduction in any medium, provided the original author(s) and source are credited.
Data Availability Statement
All relevant data are within the paper and in the Supplementary Material.
Key Words
Sections
Subjects
Authors
Author Contributions
All conceived and designed the study.
All performed the experiments/collected the data.
JWMR, CAK, PS, and RJF analyzed and interpreted the data.
All contributed resources.
All drafted or revised the manuscript.
Competing Interests
The authors have declared that no competing interests exist.
Metrics & Citations
Metrics
Other Metrics
Citations
Cite As
John W.M. Rudd, Carol A. Kelly, Patricia Sellers, Robert J. Flett, and Bruce E. Townsend. 2021. Why the English–Wabigoon river system is still polluted by mercury 57 years after its contamination. FACETS.
6: 2002-2027.
https://doi.org/10.1139/facets-2021-0093
Export Citations
If you have the appropriate software installed, you can download article citation data to the citation manager of your choice. Simply select your manager software from the list below and click Download.
Cited by
1. Near Complete Removal of Total Mercury and Methylmercury from River Water during the Production of Municipal Drinking Water
2. Characterizing visual field loss from past mercury exposure in an Indigenous riverine community (Grassy Narrows First Nation, Canada): a cluster-based approach
3. Assessment of mercury methylation and methylmercury demethylation potentials in water and sediments along the Wabigoon River system
4. Tracking mercury sources in the Wabigoon River: Use of stable mercury isotopes in bioindicator organisms
5. Spatial and seasonal patterns of mercury concentrations, methylation and demethylation in central Canadian boreal soils and stream sediment
6. The Contribution across Three Generations of Mercury Exposure to Attempted Suicide among Children and Youth in Grassy Narrows First Nation, Canada: An Intergenerational Analysis
7. Global change effects on biogeochemical mercury cycling
8. Visual Characteristics of Adults with Long-Standing History of Dietary Exposure to Mercury in Grassy Narrows First Nation, Canada