Weighing the importance of animal body size in traditional food systems
Abstract
Traditional food systems based on harvest from the local environment are fundamental to the well-being of many communities, but their security is challenged by rapid socio-ecological change. We synthesized literature and data describing how a fundamental form of biodiversity, animal body size, contributes to the security of traditional food systems through relationships with species availability, accessibility, adequacy, and use. We found larger vertebrate species were more available, accessible, and used on a per kilogram basis, particularly for mammals. Conversely, larger species were no more or less adequate from a combined nutritional, health, and cultural perspective. Larger species represented more biomass, and this biomass required less time to harvest, with greater but more variable mean caloric returns over time. Smaller species provided more consistent caloric returns and were harvested during documented shortages of prey. This reliance on species with a range of body sizes is consistent with optimal foraging theory and the evolutionary value of flexibility, and highlights the importance of a biodiverse pool of species for traditional food security in times of change. Our synthesis of published literature and data highlights the many socio-ecological correlates of species size and how these relate to the security of traditional food systems.
Introduction
Traditional food systems, or food systems based on the harvest of a diverse set of culturally acceptable species from the local environment, contribute significantly to the physical and cultural well-being of Indigenous communities in northern North America (Kuhnlein and Receveur 1996). Traditional foods remain an important source of energy, protein, and micronutrients in many communities (Lawn et al. 2002; Kuhnlein et al. 2004; Kuhnlein and Receveur 2007), and their consumption has been associated with lower rates of obesity, diabetes, and cardiovascular disease and higher rates of food security (Kuhnlein 1995; Murphy et al. 1995; Kuhnlein and Receveur 1996; Hickey et al. 2016), which is defined as “when all people, at all times, have physical and economic access to sufficient, safe and nutritious food to meet their dietary needs and food preferences for an active and healthy life” (FAO 1996). For many, the health and livelihood benefits of traditional foods are inseparable from their cultural importance (Borré 1994; Richmond and Ross 2009). When questioned, 93%–95% of Dene, Métis, Yukon First Nations, and Inuit respondents in Canada agreed that traditional foods “are an essential part of the culture here” (Lambden et al. 2007), while Resolution 98-12 of the Inuit Circumpolar Council states:
“Inuit hunting, fishing and other forms of subsistence gathering constitute a common basis of Inuit spiritual, cultural, social, economic and political way of life and are essential to the continued viability of Inuit communities and individuals” (ICC 1998, p. 68–69.).
The security of traditional food systems, that is the continued and predictable availability and access to quality and culturally acceptable foods derived from the local environment through Indigenous cultural practices (Paci et al. 2004), is being severely challenged by many forms of environmental, biological, and social change (Samson and Petty 2006; Ford 2009; Wesche and Chan 2010; Council of Canadian Academies 2014). For example, common household-reported barriers to the harvest of traditional foods include no hunter, no equipment, lack of time, lack of knowledge, restrictive regulations, and reduced availability of species (Chan et al. 2011; 2012; 2014; 2016; 2018). Food systems involve dynamic interactions between biophysical and human environments (Gregory et al. 2005), and traditional food systems are no different. Given the socio-ecological nature of traditional food systems and the challenges they face, understanding the characteristics that promote the security of traditional food systems requires integrating evidence from across social and biological disciplines.
Here we synthesized a wide range of ecological, anthropological, geographical, and food security data and literature describing a fundamental contributor to the security of traditional food systems in northern North America: body size biodiversity in animals. In northern Canada and Alaska, traditional food systems involve at least 100 wild vertebrate species spanning a wide range of body size from the 0.01 kg Alaska blackfish (Dallia pectoralis) to the 100 000 kg bowhead whale (Balaena mysticetus; Kruse 2011). The importance of species body size as a key component of biodiversity is well quantified and widely discussed in the ecological literature; body size correlates with metabolic rates, generation times, longevity, intrinsic rates of growth, range sizes, and extinction risks (Fenchel 1974; Peters 1983; Lindstedt et al. 1986; Cardillo et al. 2005; Gaillaird and Turner 2004; Speakman 2005; Burger and Ginzburg 2009). Animal body size is also widely discussed in anthropological literature as an important factor in hunter prey choice and species extinctions (Winterhalder 1981; Ripple and Valkenburgh 2010; Nagaoka 2002; Broughton et al. 2011; Grund et al. 2012). However, to our knowledge the direct importance of species size diversity to the socio-ecology of contemporary traditional food systems, and their security in particular, has not been explicitly synthesized. So, in this paper we compiled published data and literature describing how species body size relates to four aspects of the security of traditional food systems (adapted from Wesche and Chan 2010; Nunavut Food Security Coalition, 2014): (i) availability, that there are enough animals in the environment to meet needs; (ii) accessibility, that those animals can be safely reached and harvested; (iii) adequacy, that those animals are acceptable as food from a nutritional, health, and cultural perspective; and (iv) use, that those animals are harvested and consumed as traditional foods (Fig. 1). Furthermore, we briefly summarized a theoretical framework, optimal foraging theory, that has been used to interpret the role of body size in species selection in traditional food systems (McCay 1981; Stephens and Krebs 1986; Winterhalder and Lu 1997). Our goal was to draw the attention of a larger audience to body size related trends and theory and their implications for traditional food systems, as well as to give background for policy makers looking to promote the security of traditional food systems.
Fig. 1.

Methods
Author positionality and ethical considerations
All authors identify as natural scientists, most are early career researchers, and all are from the same research group at the onset of this paper. All but one of us are non-Indigenous (one self-identifies as Métis). This synthesis focuses exclusively on published materials, a medium that has historically disregarded Indigenous Knowledge. Thus, this paper presents a Western science perspective and not one rooted in Indigenous Knowledge and worldviews, offering our relatively narrow understanding of biodiversity and traditional food systems. We all have experience working in and (or) with Indigenous communities in northern North America, and these experiences inspired our search for factors that promote the security of traditional food systems facing socio-ecological change.
We recognize the continued importance of better connecting biodiversity, ecosystem services, and human well-being (Bennett et al. 2015). Traditional food systems are important links between biodiversity and well-being in certain communities, especially in remote regions like northern North America. The importance of biodiversity for traditional food systems has been demonstrated through community-based participatory research projects that engage directly with local harvesters (e.g., Guyot et al. 2006). However, based on our ecological science training and positionality, we have observed that the academic discourse on traditional food systems and their security tends to focus on studies of individual communities rather than general trends (but see Batal et al. 2021) and tends to be better connected to concepts and approaches used in health, nutrition, and social sciences rather than to fields like ecology and biodiversity (e.g., Walch et al. 2018). We wanted to provide a reference that summarized empirical and conceptual knowledge demonstrating the links between biodiversity and traditional food systems found within the published literature. As ecologists and biologists trained to focus on biodiversity writ large (and small), with a general awareness that biodiversity is dominated by smaller-bodied, unfamiliar, and often difficult to identify species, we wondered whether the contributions of smaller and less familiar species were being overlooked by the academic literature on biodiversity, ecosystem services, and traditional food systems and their security. We could not help but notice that the research priorities of our Indigenous partner organizations (e.g., Firelight and VGFN 2018; GRRB 2021) and the harvesting activities of our Indigenous colleagues repeatedly emphasized a wide diversity of traditional food species big and small.
We found the process for ethical approval of this paper difficult to define. This paper is a synthesis of published material describing social and ecological relationships of potential relevance to traditional food systems and their security. Our goal was to demonstrates the wide diversity of wildlife species—large and small, that contribute to traditional food systems—and better connect published data and concepts like allometries, scaling, and prey choice to the socio-ecology of contemporary traditional food systems and their security. Some of the quantitative relationships presented here are well established in their respective disciplines, some are not, and never have all these relationships been brought together and synthesized to our knowledge. In this format we could not do justice to the depth, complexity, and history of all the research that factors into this synthesis; instead, we strove to highlight generalities and particular examples while citing literature for interested readers to pursue for further detail. Although the importance of research that supports traditional food systems has been repeatedly emphasized (e.g., Nunavut Food Security Coalition 2014), syntheses of academic knowledge of potential relevance to traditional food systems poses a challenge for ethical oversight. We conducted no data collection, instead working exclusively with publicly available information. However, some of the material we cited includes studies conducted with various Indigenous communities spread throughout the globe. To our knowledge, this form of literature review and synthesis does not require community engagement and ethical review according to Article 9.2 of the Government of Canada’s Tri-Council Policy Statement: Ethical Conduct for Research Involving Humans (example 8; CIHR et al. 2018). While we would have preferred to engage with a group representing rights-holders in our research review, identifying the appropriate rights-holder representatives was challenging considering the global nature of literature cited. Recognizing our primarily non-Indigenous authorship, we sought out “culturally informed advice” (CIHR et al. 2018) by contacting four experts in traditional food systems for an ethical review of our manuscript following peer review but prior to publication. In adapting our manuscript in response to these reviews, our goal was to produce a reference document that minimizes the risk of causing harm.
Data sources and compilation
To review how body size variation in fish, birds, and mammals correlates with measures of species availability, accessibility, adequacy, and use, we compiled data and publications distributed in ecological, anthropological, geographical, and food security literature. Thus, this synthesis is both a review of peer-reviewed publications and a re-analysis and synthesis of published data from these fields. While traditional food systems include plants, invertebrates, and vertebrates, and size variation can be important across all three groups, we focused on vertebrates, particularly fish, birds, and mammals. These taxa include many of the species contributing to traditional food systems in northern North America and have the most readily available body size data. But they also exclude important taxa like plants, insects, invertebrates, amphibians, and reptiles (Bukkens 1997, Turner et al. 2011, Codding et al. 2014, Mendonça et al. 2014). Similarly, we focused exclusively on among species (inter-specific) body size variation because (i) species-level estimates of mean body size are more readily available than regional, population, or individual-level estimates and (ii) comparing species of vastly different body size renders results less sensitive to within species variation. Within-species size variation is also important, including regional differences associated with latitude, habitat, and subspecies or ecotype designations (James 1970, Krause et al. 1996, Blackburn et al. 1999, Couturier et al. 2010) as well as size differences among individuals present within the same population, but this intra-specific variation was not part of this synthesis. For birds and mammals, we used mean adult body mass estimates for 9 993 bird and 5 400 mammal species compiled by Wilman et al. (2014). Determining a mean species body mass is more challenging for fish than for birds and mammals, because fish are indeterminate growers and therefore defined by considerable age and resource-related size variation (Sebens 1987). For fish, we estimated mean body mass estimates of 30 246 species using FishBase (Froese and Pauly 2000; for more details see Supplementary Material 1). All data were presented at the species-level, but data sources estimating traditional food use frequently included multi-species groupings (e.g., ducks, seals, salmon, etc.). To convert these to species-level data, we divided groups equally into their component species (see Supplementary Material 2). We synthesized and visualized all data using R (R Core Team 2020).
Beyond our focus on inter-specific comparisons among fish, birds, and mammals, our synthesis focused geographically on traditional food use in northern North America (Canada and Alaska), as use has been well-documented (e.g., AMAP 2018) in this region. However, many of the body size correlations presented here that are relevant to the availability, accessibility, and adequacy of traditional food species represent global data sets. These global correlations are intended to emphasize the generality of body size patterns, while avoiding the ecosystem and taxonomic exclusions introduced by regionalized data (e.g., excluding marine fish and mammals from continental data sets, migratory birds from breeding bird inventories). In addition, the predictive strength of size relationships generally increases with the range of body sizes being compared.
Body size and the availability of traditional foods
The availability of traditional foods is influenced by many ecological traits that scale with body size like species diversity, abundance, productivity (i.e., births and deaths), and population stability. The allometric relationships between these traits and mean species body size have been well documented by ecologists (Peters 1983; Calder 1996; Brown and West 2000). There are more species of small animals than large animals. Among vertebrates, across continental to global scales, the relationship between species diversity and vertebrate body mass is log normally distributed with the highest diversity occurring at approximately 0.1 kg (Fig. 2; Kozlowski and Gawelczyk 2002; Burger and Ginzburg 2009) and a slightly fatter tail of larger body masses to the right of the mode (Kozlowski and Gawelczyk 2002; Clauset 2013). Very large and very small vertebrates are both characterized by lower diversity, but the size range above the 0.1 kg mode is a lot larger (e.g., up to a 100 000 kg blue whale; Balaenoptera musculus) than the size range below (e.g., down to a 0.01 kg Alaskan blackfish). This size-diversity distribution is believed to result from an evolutionary trade-off between short-term advantages of larger body mass (e.g., greater fecundity, resource monopolization, tolerance of resource fluctuations, survival, thermoregulation) being counterbalanced by a greater risk of extinction due to longer generation times, lower intrinsic rates of growth, lower densities, and larger range requirements (Blackenhorn 2000; Cardillo et al. 2005; Clauset and Erwin 2008; Burger and Ginzburg 2009; Raia et al. 2012; Clauset 2013).
Fig. 2.

Larger traditional food species tend to occur at lower densities but represent greater biomass on the landscape than smaller species. The pattern in density, or individuals per unit area, is intuitive. Because larger-bodied individuals occupy more space and require more resources than smaller-bodied individuals, populations of larger-bodied species should be composed of fewer individuals per unit area than smaller-bodied species (Peters and Wassenberg 1983; Waguespack and Surovell 2003; White et al. 2007). In contrast it appears that overall biomass, or kilogram per unit area, generally increases with species body size. Larger traditional food species, and especially larger species that happen to be locally abundant, tend to represent greater quantities of biomass in the wild (Fig. 3; Peters and Wassenberg 1983; Peres 2000; but see Winterhalder 1981) even though smaller species tend to be more numerous in terms of individuals per area than larger species.
Fig. 3.

Smaller traditional food species tend to grow and reproduce more quickly on a per gram basis than larger species. Put more precisely, the relationship between annual biomass production (i.e., growth and reproduction) and body size in vertebrates has an exponent approximating 0.75 (Ernest et al. 2003). Energy investment in growth and reproduction detracts from investment in maintenance; consequently smaller organisms generally have shorter lifespans (Roff 1992; Stearns 1992). Thus, relative to larger organisms, smaller traditional food species tend to live fast and die young. This life history pattern is often expressed in relation to the intrinsic rate of increase for a species, rmax, a parameter used in population dynamic equations to reflect the capacity of a population to grow in typically favourable, unconstrained conditions (Coulson and Godfray 2007; Cortés 2016). Plotting empirically derived estimates of rmax as a function of body mass yields a clear pattern: smaller species have a higher intrinsic rate of increase than larger species (Fig. 4). This higher-per-capita production is directly relevant to the harvest of traditional foods, since sustainable yields depend more on the annual production of a population rather than on its overall density (Fryxell et al. 2014). As long as annual harvest does not exceed annual production, at least in theory, a traditional food species can be harvested in perpetuity without causing a decline (Weinbaum et al. 2013). Since larger species are typically characterized by lower-per-capita production, sustainable harvest rates (as a percentage of population size) will generally decline with body size. Furthermore, smaller species will tend to rebound from episodes of over-harvest faster than larger species (Frank et al. 2011).
Fig. 4.

Populations of smaller species with higher intrinsic rates of increase are also more likely to be characterized by population instability, including oscillatory, cyclic, and chaotic dynamics, than slower-growing populations (May 1974; Bonsall and Hassell 2007). All else being equal, the population size of smaller traditional food species will tend to fluctuate faster and more than the population size of larger species. This tendency towards faster and greater fluctuations can be expressed, formally, as differences in the period and amplitude of population oscillations. Among birds and mammals characterized by regular fluctuations in abundance, periodicity of population cycles scales positively with body size, ranging from approximately 3 years for species weighing 0.01 kg to approximately 30 years for species weighing 100 kg (Peterson et al. 1984; Myers 2018). The tendency for smaller species to be characterized by larger amplitude fluctuations in population size than larger species is apparent from population time series made available by the global population dynamics database for many of the world’s fish and mammal populations (although less so for birds; Fig. 5; NERC Centre for Population Biology 2010).
Fig. 5.

Body size and the accessibility of traditional foods
In the context of food systems, accessibility generally includes physical, social, and economic access to food (Barrett 2010). For traditional food systems, we considered physical access to be the distances traveled and time needed to acquire traditional foods; social access the knowledge, group cooperation techniques, and regulatory frameworks (or lack thereof) needed to acquire traditional foods; and economic access the capital and operational costs borne to acquire traditional foods.
Regarding physical access, search and handling times needed to find, harvest, and process traditional foods tended to increase with species body size. Search times have traditionally been viewed as the largest time expenditure associated with harvesting (Winterhalder 1981; Lupo and Schmitt 2016) and, since animal densities (individuals/km2) tend to decline with body size (see availability section), search times tend to increase with size (Simms 1987; Byers and Ugan, 2005; Lupo and Schmitt 2016). Similarly, handling times tend to increase with body size, and this increase can be subdivided into longer pursuit times, declining hunting success, longer processing times, and greater transportation times (O’Connell et al. 1988; Lupo 2006; Lupo and Schmitt 2016). Nonetheless, the bigger bodies of larger species more than compensate for the longer handling times they require, meaning the general trend is for average post-encounter return rates (energetic returns/handling time) to increase with body size (Fig. 6; Broughton et. al. 2011; Ugan 2005; but see Bird et al. 2009). Despite this average trend, it should be noted that there can be considerable variability in species return rates depending on a variety of factors including the season, prey characteristics and nutritional condition (see adequacy section), tools, and harvester group composition, such that smaller animals can be highly profitable under the right circumstances (e.g., harvested en masse like in spawning fish aggregations, rapidly processed like when eaten whole; Churchill 1993; Ugan 2005; Bird et al. 2013; Lupo and Schmitt 2016). These conditions could explain some of the smaller species with very high potential return rates (e.g., the 0.28 kg tui chub (Siphateles bicolor; Lindstrom 1992), the 1.5 kg cutthroat trout (Oncorhynchus clarkia; Ugan 2005)). Also, at the largest end of the body size spectrum (e.g., the 3 940 kg African bush elephant (Loxodonta africana)), post-encounter return rates could decline as a result of rapid increases in all forms of handling times (Ugan 2005; Lupo and Schmitt 2016). Finally, while overall average returns increase with body size, so does the average variability of those returns (note the logarithmic y axis in Fig. 6; Hawkes et al. 1991; Lupo and Schmitt 2016). So, while the harvest of larger species typically increases the physical accessibility of traditional foods, it can also increase the unpredictability of those returns.
Fig. 6.

Harvesters may manage the variable returns of larger species through cooperation, division of labour, and knowledge sharing between harvesters, i.e., social techniques for moderating food accessibility. The harvest and processing of larger species generally takes more time, is more technically challenging, and can be more dangerous; this is particularly the case for extremely large species (e.g., elephants, whales; Condon et al. 1995, Smith 1991; Alvard 2002; Lupo and Schmitt 2016). Thus larger species can require more knowledge and expertise to harvest and process, a trend reflected in greater rates of hunting failure for larger species (Lupo and Schmitt 2016) and the tendency for occasional, generally less experienced, harvesters to focus on smaller (e.g., waterfowl, fishing) rather than larger species (e.g., caribou (Rangifer tarandus), polar bear (Ursus maritimus), muskox (Ovibos moschatus); Condon et al. 1995). Since finding larger species typically requires longer search times, knowledge of environmental conditions for travel, safety, and equipment repair is critical for accessing larger game, especially for large marine mammals (Cummins 1992; Condon et al. 1995, Rosol et al. 2016, Usher 2002; Pal et al. 2013). The variability of harvesting larger species may be further offset by nonmeat foraging, food storage, and food sharing (Kaplan and Gurven 2005; Gurven and Hill 2009). When labour is divided between genders, men often pursue large game while women forage for nonmeat foods and small animals; big game hunting often increases if women’s foraging returns decline. (Bousman 1993; Gurven and Hill 2009). Devoting labour towards food processing (e.g., drying, the preparation of bone grease) and storage allows for larger game to be collected in higher quantities when seasonally accessible and preserved for times of scarcity (Binford 1978; Bettinger et al. 2015). Larger species are also more frequently shared outside of the immediate family compared with small game, plants, or other nonmeat resources (Kaplan and Gurven 2005). These cooperative strategies do not just manage the variability of harvest returns of large game, they are often integral to building social relationships and status within families and communities (Kishigami 2000; Grete 2000; Alvard 2002). Finally, social accessibility of traditional foods is also affected by local rule frameworks like land tenure, public safety laws, and wildlife harvest regulations. Larger species are more likely to be designated at-risk and to be subject to some kind of harvest restrictions (Cardillo et al. 2005; Schipper et al. 2008), restrictions that often become the subject of legal disputes. For example, the number of decisions rendered by the Supreme Court of Canada regarding Indigenous harvest rights tends to increase with the body size of the species in question (Fig. S1, Supplementary Material 3).
Economic barriers (e.g., the capital and operating costs of harvesting) can also limit access to traditional foods. The initial capital investments to obtain hunting and fishing equipment can be an insurmountable barrier for low-income families (Condon et al. 1995; Nelson et al. 2005; Chan et al. 2011). Operating costs, particularly fuels, can also be a major impediment to harvest (Lambden et al. 2006; Pal et al. 2013; Randazzo and Robidoux 2018). Although accounts of harvesting costs by species are rare, Usher (1971) recorded 14 months of capital and operational costs of Inuit harvesters in Sachs Harbour, Canada, alongside target species and output. He calculated production costs per animal as a combination of the depreciation of capital goods used in harvest as well as the operational costs to engage in harvesting. Usher (1971) found that overall production costs per animal tended to increase with body size (Fig. 7a), because larger animals typically required more expensive equipment (e.g., boats, higher calibre rifles, and ammunition) and larger travel distances. However, this trend of increasing production costs with body size is lost when considering costs per kilogram of edible tissue acquired (Fig. 7b). Note that Usher’s (1971) analysis preceded the transition from dog teams to snowmobiles for winter transportation, and this could increase both the capital and operating costs of the winter travel needed to harvest larger species like polar bear and caribou (although a more recent analysis including snowmobile costs still showed no relationship between production costs per kilogram and body size; Randazzo and Robidoux 2018). Interestingly, in Amazonia, dramatic increases in ammunition costs were associated with a shift to greater harvesting of larger species (Sirén and Wilkie 2016), suggesting that in certain contexts economic costs can influence traditional harvesting practices.
Fig. 7.

Body size and the adequacy of traditional foods
According to the United Nations’ Special Rapporteur on the right to food, food adequacy “requires that food satisfy dietary needs... be safe for human consumption, free of adverse substances, and culturally acceptable” (de Schutter 2010, p. 4). As such, we reviewed how the body size of traditional food species correlates with their adequacy in terms of their nutritional density and body composition, lack of chemical contamination, and cultural acceptability.
Traditional foods typically contain higher levels of protein, lower levels of fat, and are considered healthier than their market alternatives (Kuhnlein et al. 2009; Lambden et al. 2007). However, there is considerable variation in body composition and nutritional content of different species’ tissues (Rosol et al. 2016). In all taxa, the proportion of animal bodies that is muscle, an important edible tissue, stays relatively constant across body sizes except in extremely large mammals (i.e., >1 000 kg; Fig. 8). In mammals and birds, increases in body size result in fat and structural components like bone accounting for a larger fraction of total mass while skin, integument, and organs account for a smaller fraction (Figs. 8a and 8c). Declines in the proportion of skin and integument in larger mammals and birds reflects the declining surface area to volume ratio, while declines in overall organ mass with body size reflect lower metabolic demands on a per kilogram basis. Bone mass increases disproportionately with body size due to a required thickening of long bones to resist the additional forces associated with larger body masses (Prange et al. 1979; Biewener 1989; 1990). While typically not a major source of calories, bones, especially the marrow of long bones, provide a noteworthy source of fat particularly when prey are lean (e.g., during seasonal resource shortages; Speth 1989). Though edible fractions (muscle, fat, organs) of birds and mammals, and to a lesser degree fish, tend to decline with body size (Smith 1991; Byers and Ugan 2005; Lupo and Schmitt 2016), the concentrations of calories, protein, and fat within muscle and organ tissue show no strong relationship with body size (Supplementary Material 4; Kuhnlein and Humphries 2017). Only in birds does the protein content of muscle tissue decrease with body size (Fig. S2c, Supplementary Material 4), potentially due to an increase in muscle fat content (Fig. S2b, Supplementary Material 4). Overall larger mammals and birds should still be more calorically dense primarily due to larger fat stores. In contrast, organs account for a smaller proportion of total mass in larger birds and mammals. Organs like the liver are often associated with higher concentrations of micronutrients, like iron and vitamin A, so declining organ fractions could result in reduced micronutrient concentrations in larger species. This trend could be magnified (e.g., iron; Fig. S3j, Supplementary Material 4) or reduced (e.g., vitamin A; Fig. S3l, Supplementary Material 4) by changing micronutrient concentrations with body size in organ tissues, but generally it appears changing tissue proportions, rather than tissue content, drive changing nutritional patterns with body size (Smith 1991).
Fig. 8.

An important component of traditional food adequacy is that species remain “free from adverse substances” (de Schutter 2010). Both natural and anthropogenically sourced contaminants in traditional foods have been a growing interest and concern in many Indigenous communities (Pirkle et al. 2016; Wheatley and Paradis 1995). Larger traditional food species, particularly marine mammals at higher trophic levels, tend to contain higher concentrations of certain contaminants. Many factors play a role in driving inter-individual, inter-population, and inter-specific variation in tissue contaminant concentrations in wild foods (e.g., location, habitat, trophic position, diet, sex, age, breeding status, ecological change; Barst et al. 2019; Provencher et al. 2010; McKinney et al. 2009; Ross et al. 2000). Despite these numerous confounding factors, when compared across species and tissue types, population-level mean and median concentrations of total mercury and persistent organic pollutants like polychlorinated biphenyls (PCBs) and dichlorodiphenyltrichloroethane (DDT) do tend to increase with body size (Fig. 9; Supplementary Material 5; NCP 2003; 2012; 2013; AMAP 2018). Total mercury, for example, tends to increase with body size across nonherbivorous species, likely as a result of biomagnification given larger nonherbivores tend to be at higher trophic levels (Fig. 9b; Kelly et al. 2007). It is noteworthy, however, that methylmercury, the form of mercury that readily biomagnifies, is produced mainly in aquatic systems and is typically lower in terrestrial systems (UNEP 2019). All the herbivorous species included in this synthesis are terrestrial, which may explain why biomagnification was not observed at this trophic level (Fig. 9b).
Fig. 9.

The cultural acceptability of, or preferences for, particular traditional foods is highly context- and culture-dependent (Garibaldi and Turner 2004); similarly, the relationship between species body size and cultural preferences, if any, varies considerably by community. Traditional foods can be subject to social restraints, and these restraints can take many forms such as the protection of entire biological communities, habitat patches, or particular species (Berkes et al 1995). Colding and Folke (1997) documented 55 such social constraints around particular traditional food species, and found that these species tended to be larger, particularly larger mammals (Colding and Folke 1997; Fig. S5, Supplementary Material 6). These social restraints existed for a wide variety of reasons, including a species’ behavioural or morphological characteristics, a perception of the species as toxic, its role in local cosmology, or as a conservation mechanism (Colding and Folke 1997). Similar to outright harvesting restrictions, cultural preferences for species can show a body size pattern. For example, in some communities of northern Canada larger animals, such as caribou or black bear (Ursus americanus), can be perceived as more spiritually powerful and of greater nutritional value, thus making them “stronger foods”. Hunters that harvested these larger species can have a greater social status, and Elders are offered these species as a sign of respect. The concept of well-being, or “being alive well”, involved the consumption of a diversity of traditional foods, particularly these “stronger” species (Adelson 1992). This is not to suggest that smaller traditional food species were inadequate. Instead, this is a case of species cultural preferences, necessarily intertwined with economic and nutritional preferences, coinciding to a degree with species body size (Adelson 1992; Koster et al. 2010; see also Hames and Vickers 1982). In other contexts, or when comparing across cultures, body size patterns in species preferences may change. Comparing the traditional food consumption patterns of communities occupying the same town site notionally eliminates the role of distinct geographic homelands changing the availability and accessibility of traditional food species. In one such community in northern Canada (Supplementary Material 7) one group of harvesters preferentially consumed larger, generally marine, mammals, while the other preferentially consumed terrestrial species, often smaller birds and fish (JBNQ NHRC 1982, 1988).
Body size and the use of traditional foods
Estimating the use of traditional food species across body sizes is challenged by the variety of data sources, the many ways to quantify use, and the subjectivity of any metric of use (Usher and Wenzel, 1987). We quantified traditional food use in northern North America via compilations of three independent, multi-year, data sets originating in northern North America: food recall questionnaires, wildlife harvest surveys, and zooarcheological archives. For each, we standardized data from multiple communities, regions, or sites at the species or species-group level (e.g., “geese”) by converting data with variable units into proportions of the total for that location in that study (e.g., lake whitefish Coregonus clupeaformis represent 7% (i.e., 0.07) of reported traditional food consumption in Eastern Hudson Bay; Lemire et al. 2015). For each species we calculated a mean proportion value across communities or regions so that each species’ “use score” ranges between 0 and 1 and the scores of all species sum to 1 (Supplementary Material 8). We applied these use scores as weights in kernel density estimates so that species used more frequently contributed more to probability density functions than species used infrequently. Plots of probability density functions visualize how the intensity of use of traditional food species varied across body size. For comparison, the unweighted probability density function of all Canadian species is in the background (Fig. 10; Supplementary Material 8).
Fig. 10.

All use data sets produced surprisingly similar probability density functions; the use of traditional foods placed greater emphasis on larger animals, and particularly larger mammals, compared with what was available (Figs. 10a–10i). The densities of body sizes used typically peaked at species that were up to several thousand times heavier than the most common body sizes of Canadian vertebrate species. Birds were the least used taxon, representing between 2% and 16% of the species used in each data set while representing 34 % of species in Canada (Figs. 10a, 10d, and 10g). The most frequently used bird species ranged from the 0.5 kg spruce grouse (Falcipennis canadensis) to the 2.8 kg Canada goose (Branta canadensis), 20–100 times heavier than the size of bird species most commonly available in Canada (around 0.025 kg). But bird diversity in Canada is bimodal and the second availability peak at 0.6 kg more closely coincided with what was used. Birds were particularly underrepresented in the harvest surveys (Fig. 10g). Fish were used in a relatively similar proportion (38%–52%) to their overall diversity (55% of species in Canada) with the most frequently used species having body sizes between the 1.5 kg cutthroat trout and the 2.3 kg turbot (Scophthalmus maximus; Figs. 10b, 10e, and 10h), up to 13 times larger than the most commonly available fish species (∼0.18 kg). The zooarchaeological data were a notable outlier (Fig. 10b), as they contained a greater breadth of fish sizes that more closely resembled what was available with two peaks between the 0.15 kg buffalo sculpin (Enophrys bison) and the 1.6 kg sockeye salmon (Oncorhynchus nerka). Finally, mammals were used as traditional foods (39%–46%) far beyond their available diversity (10% of species in Canada) with the most frequently used species having body sizes between the 100 kg black bear (Ursus americanus) and the 130 kg harp seal (Pagophilus groenlandicus; Figs. 10c, 10f, and 10i). The most frequently used mammal species were up to 5 000 times heavier than the body sizes most commonly available in Canadian mammals (shrew-sized species ∼0.028 kg), but mammal diversity in Canada is also bimodal with a second peak at 100 kg. Interestingly, the overall use of fish and mammals was similar, even though Canadian mammal diversity (213 species) is far less than fish (1126 freshwater and marine species).
Discussion
Here we synthesized academic data and literature describing how a fundamental component of biodiversity, species body size, correlates with a variety of socio-ecological characteristics that could influence the availability, accessibility, adequacy, and use of wild vertebrates in traditional food systems. Compiling data sets from traditional food systems in northern North America, we found larger species, particularly larger mammals, were more frequently used compared with smaller species, particularly birds (Fig. 10). These results were consistent across three independent data sets quantifying use: food recall questionnaires, harvest surveys, and archaeological inventories, even though each data set had different weaknesses when estimating traditional food use. Food recall questionnaires typically reflect more recent meals, even when respondents are asked to describe consumption over longer time frames. As such, they can reflect the seasonal and short-term availability of traditional food species depending on the timing of the survey (Joachim 1998). Some studies repeat questionnaires throughout a calendar year or have participants keep a diary to improve estimates of annual consumption patterns (Mackey 1988; Morrison et al. 1995; Wein and Freeman 1995). Despite these concerns and others (e.g., nonrepresentative sampling), food recall questionnaires remain the most direct quantitative estimates available of traditional food use. In contrast, harvest surveys are typically conducted to inform wildlife management, so they include species harvested for nonfood purposes (e.g., furbearers) and will focus on species of greater management concern. Frequently subsistence harvest, in particular fish and smaller game, is underreported (Usher and Wenzel 1987); this could explain the lower incidence of birds (Fig. 10g) and the narrower peaks for fish and mammals (Figs. 10h and 10i) in harvest density distributions. Still, because of the importance of harvest data for wildlife management and Indigenous land claims, harvest data are by far the most extensive estimates of traditional food use with 631 community-year records included in this synthesis (Kruse 2011; 2017). Finally, the relative frequency of identifiable specimens at archaeological sites is our least direct estimate of traditional food use because: (i) not all animals consumed are necessarily transported to any particular site; (ii) site use varies seasonally; (iii) taphonomic factors (e.g., scavengers, decomposition) are known to affect the remains of smaller species, and birds, disproportionately; (iv) sites may be discovered or selected for detailed analysis based on the presence of larger animal remains; and (v) archaeological specimens, if they reflect harvesting activities, reflect harvesting that has occurred over much longer time frames than food recall and harvest surveys (Binford 1980; Outram 2001; Surovell and Waguespack 2009). Considering these potential sources of variation, the body size distribution of specimens in our archaeological inventories is remarkably similar to that of food recall and harvest surveys, the most notable difference being a relatively greater frequency of smaller fish species (Fig. 10b; Surovell and Waguespack 2009). Each data set, regardless of their distinct and different origins, show a greater frequency of larger species, particularly mammals, than would be expected from the simple vertebrate diversity distributions in northern North America.
Larger species also shared characteristics associated with greater availability and accessibility as traditional foods. Availability increasing with larger species may appear counterintuitive; larger species are generally less diverse (Fig. 2), numerous (White et al. 2007), and productive (Fig. 4) than smaller species, making them slower to recover from harvest (Frank et al. 2011) and more prone to extinction (Gaston and Blackburn 1995; Cardillo et al. 2005; Olden et al. 2007). However, larger species offer more biomass on the landscape (Fig. 3; Peres 2000), particularly during congregations of individuals (e.g., mass migrations). Still, during shortages of larger species, the higher diversity of smaller traditional food species results in a greater selection to fall back upon. This is the case even though smaller species tend to experience rapid population fluctuations (Fig. 5), and there are many reports of harvesters turning to smaller traditional foods when larger species are unavailable (Rogers and Black 1976; Vuntut Gwitchin First Nation and Smith 2009).
Larger food species are not just more biomass on the landscape; this biomass tends to be more accessible to harvesters. Larger animals tend to contain more calories that require less time to harvest, an energy richness that is partially driven by greater proportions of fat in larger mammals and birds (Fig. 8). While the amount of time needed to pursue and process game increases with body size, caloric densities tend to increase more rapidly leading to greater mean caloric returns per unit time in larger animals (Fig. 6). Note, however, that species returns can vary significantly depending on the circumstances, that the variability of returns also increases with body size, and the trend of increasing returns with body size may reverse for extremely large species, particularly when terrestrial (Nagaoka 2002) due to increased pursuit and transportation costs (Smith 1991; Lupo and Schmitt 2016). While larger species are often more challenging to harvest, process, and transport (Condon et al. 1995; Lupo and Schmitt 2016), the use of cooperative techniques (e.g., food sharing, food storage, cooperative hunting) can manage these challenges (Binford 1978; Kaplan and Gurven 2005). The economic accessibility of species, at first glance, also appears to decline with body size (i.e., costs increase; Fig. 7a), but when calculated per kilogram biomass this decline disappears (Fig 7b; Usher 1971). Important to note that this trend is based on a single community (Usher 1971), that significant interspecific variability exists in these cost estimates and that more data are required to accurately describe how economic accessibility of traditional food species varies with body size in different contexts. For example, in eastern Ecuador Sirén and Wilkie (2016) found that a 300% increase in taxes on ammunition was associated with a dramatic decline in the harvest of smaller species that provided less biomass per shot. In contrast, harvester surveys from other regions of North America have reported time, more than cost, as the most frequent barrier to accessing traditional foods, particularly for those working wage jobs (Nelson et al. 2005; Natcher et al. 2016). Given these reports, it is noteworthy that food recall and harvest surveys in northern North America report a disproportionate use of larger species (Figs. 10d–10i), species that often maximize caloric returns per time spent.
While data sets describing species availability, accessibility, and use often demonstrated increases for larger traditional food species, adequacy data did not vary consistently with body size. Nutritionally speaking, the case has been made that switching between the tissues of different species can alter macro or micronutrient intake (e.g., Rosol et al. 2016), but these inter-specific differences did not show a strong relationship with body size (Fig S2, S3, Supplementary Materials 4). We did find tissue compositions of animals changed consistently with body size (Fig. 8; Calder 1996); this likely has greater nutritional consequences with larger animals typically containing more fat and less organ tissue. This increase in fat in larger species occurs in both fat tissue deposits and bone marrow (Speth 1989; Thompson et al. 2019) such that eating exclusively animals with insufficient fat, often smaller species, can cause repeated nausea and diarrhea, or “rabbit starvation syndrome”, with potentially lethal consequences (Speth and Spielmann 1983; Bilsborough and Mann 2006). Yet many contaminants, including mercury (Fig. 9), DDT, and PCB, (Supplementary Material 5, Fig. S4) also tended to increase in concentration with body size, suggesting the adequacy of nutritionally desirable larger species could be reduced due to contamination. Manifestations of this trend include risk assessments encouraging the substitution of beluga (Delphinapterus leucas) with smaller species to reduce PCB intake (Duhaime et al. 2004). Larger species were also more often the focus of harvesting taboos (Supplementary Material 6, Fig. S5; Colding and Folke 1997), but also were culturally preferred in certain contexts (Adelson 1998). For the most part, traditional food species are by definition culturally adequate and preferences reflect a culture’s tendency to consume a species more frequently rather than absolute prohibitions (Adelson 1998; Koster et al. 2010).
A substantial body of research has studied prey selection choices using the conceptual framework of optimal foraging theory, specifically diet breadth models (Smith et al. 1983; Stephens and Krebs 1986; Winterhalder and Lu 1997; Koster et al. 2010; Hansen et al. 2013). This framework describes the prey selection choices of a hypothetical harvester (e.g., hunter, fisher, trapper, forager) who decides whether to harvest a species based on optimizing a particular currency or utility (e.g., kilogram of meat/money spent, calories of meat/time spent; Usher 1971; Rapport and Turner 1977; Winterhalder 1987; Pal et al. 2013; Natcher et al. 2016; Randazzo and Robidoux 2018). Prey species are ranked according to currency returns, often post-encounter net calories over time, that they provide, and species are only harvested if they are part of the set of species that, combined, maximize currency returns. Based on the assumptions of the diet breadth model, an optimal harvester will preferentially harvest a higher-ranked over a lower-ranked species if they are encountered together (Winterhalder 1987; Condon et al. 1995). When lower-ranked species are encountered independently, they are only harvested when their currency returns are greater than the returns after searching for and then handling higher-ranked species (Winterhalder 1987). Put another way, a hypothetically optimal harvester will decide whether to harvest an animal based on how long it would take to find a preferred alternative (for more detailed descriptions see Smith et al. 1983; Stephens and Krebs 1986; Winterhalder 1987; Bettinger et al. 2015). It is important to remember that this conceptual framework describes a hypothetical scenario (e.g., a harvester who searches for all prey simultaneously; who makes decisions based on a single criterion, maximizing currency returns; who always has full knowledge of the exact returns associated with each species; etc.) that, much like a frictionless engine, is a measuring stick against which to compare rather than an accurate description of the real world. With this in mind, the tendency of larger species to provide greater post-encounter caloric returns (Fig. 6; Broughton et al. 2011) suggests they would rank highly for a harvester optimizing calories for time spent. While there exists significant variability in the body size – energetic returns relationship (e.g., Bird et al. 2009), the correlation is strong enough, and alternative metrics are sufficiently lacking, that body size is often used as a rough proxy for energetic returns in archaeological analyses of harvesting (Broughton et al. 2011; Bettinger et al. 2015). The implications of this ranking based on body size include that, for an optimal harvester, diet breadth narrows (i.e., lower-ranked, often smaller, species are overlooked) when search times of higher-ranked, frequently larger species are reduced (e.g., they are more numerous or foragers are more mobile) and widens when search times are increased (e.g., high-ranking prey are less numerous; Rogers and Black 1976; difficult travel conditions, Cummins 1992, Winterhalder 1981). Most importantly, variations of the diet breadth model (Winterhalder 1986), similar to other conceptual frameworks like the evolutionary value of flexibility (McCay 1981), predict that by flexibly including or ignoring species depending on the circumstances, optimal harvesters maintain a more consistent and secure intake of traditional foods even during dramatic fluctuations in the availability and accessibility of preferred species.
This link between flexibility and security is an important nuance, and it is underemphasized in our results that highlight a static, optimized, relationship between species use, availability, accessibility, and body size. Our data sets describing the use of traditional foods contain entries for 298 unique vertebrate species in northern North America. This extraordinary biodiversity is the biological foundation for flexibility in traditional food systems in this region, and this only represents vertebrates. The additions of plants, fungi, and invertebrates complete the biodiverse foundation of food systems. There are many documented instances of the flexible use of local biodiversity to adapt to changing socio-ecological circumstances (Table 1; Hayden 1972; Rogers and Black 1976; Hames and Vickers 1982; Berkes and Jolly 2001; Nagaoka 2002; Broughton et al. 2011; Berbesque et al. 2014). We were not able to empirically represent this flexible use of biodiversity in this review, but that is not to suggest that flexibility in traditional food systems is unimportant. Many of the empirical patterns summarized here are species-level averages instead of measures of variability that reflects our, and to a degree the cited literature’s, bias towards optimality rather than flexibility. This reflects our positionality (see Methods), as well as the Western and scientific background of much of our cited literature. Canada’s Royal Commission on Aboriginal Peoples (1993) has pointed out that the existing body of research, particularly research regarding Indigenous Peoples, must be open to reassessment considering its frequent lack of inclusion of Indigenous Knowledge holders. The data and theory synthesized in this paper must be considered in this light. This is not a fulsome review of all human knowledge regarding biodiversity’s contributions to traditional food systems, but instead a synthesis of primarily non-Indigenous scholarship of potential relevance to the security of traditional food systems.
Table 1.
Location | Time frame (years CE) | Socio-ecological change | Harvest focus before (mass in kg) | Harvest focus after (mass in kg) | Reference |
---|---|---|---|---|---|
Arctic North America | 1000–1850 | Climate cooling | Bowhead whales (100 000) | Caribou (90), ringed seal (70), Arctic char (0.7) | Wenzel (2009) |
Nunavut | 1940–2008 | Climate warming | Ringed seal (70) | Canada geese (2.8), snow geese (2.6), Arctic char (0.7), Greenland cod (19), shorthorn sculpin (0.2) | Imrie (2009) |
Alaska–Yukon | 500–1850 | Climate warming, increased fires | Caribou (70) | Moose (360) | Yesner 1989 |
Alaska–Yukon | 500–1850 | Habitat change from interior to coastal | Caribou (70) | Snowshoe hare (1.7), Pacific salmon (1.5–4.6), squirrels (0.2–0.8), hoary marmots (7), beaver (20), porcupine (7), muskrat (1), otter (8) | Yesner (1989) |
Prince of Wales Island, Alaska | 1970s–2020 | Logging | Pacific salmon (1.5–4.6) | Black-tailed deer (50) | Brinkman et al. (2007) |
Northern Ontario | Late 1700s–1920 | Moose and caribou declines | Moose (360), caribou (70) | Snowshoe hare (1.7), walleye (1.3), lake whitefish (1.3), suckers (0.1–0.7), sturgeon (5.5), lake trout (1.1), pike (0.4) | Rogers and Black (1976) |
Ecuador | 1973–1979 | Prey declines | Tapir (300), peccaries (32), primates (3–4) | Paca (8.2), armadillo (1.5–4.4), agouti (2.7), coatimundi (3.8–4.0), squirrel (0.7), tin-tin (0.6–1.0) | Hames and Vickers (1982) |
Emphasising the importance of biodiversity to the security of traditional food systems is made all the more urgent by the speed of socio-ecological changes being experienced by many communities (e.g., in northern North America: Guyot et al. 2006; Ford 2009; Royer and Hermann 2011; Ford and Pearce 2012; Council of Canadian Academies 2014). Here we synthesized data and publications that describe the numerous socio-ecological correlates of a fundamental component of biodiversity and mean species body size and how these influence the availability, accessibility, adequacy, and use of traditional foods. Broadly speaking, we found larger traditional food species represented digestible biomass that was more available and accessible and, particularly with mammals, more frequently used. Conversely, smaller species, although less available and accessible on a per kilogram basis, were more consistently available as they were more diverse and recovered more rapidly from harvest. All while being no more or less consistently adequate than larger species. Put more succinctly, while larger traditional food species were often more rewarding to harvest, smaller species were often more resilient. It is important to keep in mind that the use patterns described here are particular to northern North America, use patterns elsewhere may differ, use presented here is static when in reality it is highly dynamic, and traditional food species are harvested for many reasons beyond their use as food (e.g., textiles, tools, ceremony, worldview, medicine; Garibaldi and Turner 2004). Our focus here on body size and its implications should not be taken to suggest that size-independent determinants of traditional food security are unimportant. Species body size is only one of a vast number of biodiversity traits relevant to their socio-ecology, and traditional food use is influenced by many other factors (e.g., harvester wealth, employment status, preferences, knowledge, physical health, equipment, social network, regulations, seasons, competition, trading and sharing; Nelson et al. 2005; Delormier et al. 2009; Natcher et al. 2016; Batal et al. 2021). Nonetheless, there is evidence that scaling relationships play an important role in many socio-ecological systems (West 2017), and our synthesis demonstrates the breadth of characteristics correlated with species body size in traditional food systems. Theoretical frameworks like optimal foraging models (Winterhalder 1986) and the evolutionary value of flexibility (McCay 1981), alongside empirical evidence from ethnographic, archaeological, and historical accounts (Table 1), suggest that access to a wide range of species with a diversity of ecological and sociological characteristics can contribute to the security of traditional food systems. Keeping in mind that this review could only considers published material, much of which does not explicitly consider Indigenous Knowledge, we hope it could still demonstrate the very tangible connections between biodiverse pools of wild species and the security of traditional food systems.
Acknowledgements
The authors would like to thank Joanna Griffin for her support with data gathering and analysis, as well as Bruce Winterhalder, John Chételat, Duncan W. Warltier, Sonia Perillat-Amedee, Birgit Braune, Robert Letcher, Neil Burgess, Aaron Fisk, and Derek Muir for contributing material to this review. The authors would also like to acknowledge funding support from the Garfield Weston Foundation and the National Sciences and Engineering Research Council of Canada. The authors would like to voice our respect for the many Indigenous Nations that have hosted us in our studies, particularly those that host McGill University including the Haudenosaunee and Anishinabeg nations. Finally the authors would like to express our deep gratitude to Faye and the late and dearly missed Earl Benjamin. Your snare lines and soups were the inspiration for this synthesis. Mahsi’!
References
Adelson N. 1992. “Being Alive Well”: Indigenous Belief as Opposition among the Whapmagoostui Cree. Ph.D. thesis, McGill University, Montreal, Quebec. 269 p.
Adelson N. 1998. Health beliefs and the politics of Cree well-being. Health, 2(1): 5–22.
Alvard MS. 1993. Testing the “ecological noble savage” hypothesis: Interspecific prey choice by Piro hunters of Amazonian Peru. Human Ecology, 21(4): 355–387.
Alvard MS. 2002. Carcass ownership and meat distribution by big-game cooperative hunters. Research in Economic Anthropology, 21: 99–131.
AMAP. 2018. AMAP Assessment 2018: Biological Effects of Contaminants on Arctic Wildlife and Fish. Arctic Monitoring and Assessment Programme (AMAP), Tromsø, Norway. 84 p.
Barrett CB. 2010. Measuring Food Insecurity. Science, 327: 825–828.
Barst BD, Drevnick PE, Muir DC, Gantner N, Power M, Köck G, et al. 2019. Screening-level risk assessment of methylmercury for non-anadromous Arctic char (Salvelinus alpinus). Environmental Toxicology and Chemistry, 38(3): 489–502.
Batal, M, Chan HM, Fediuk K, Ing A, Berti P, Sadik T, and Johnson-Down L. 2021. Importance of the traditional food systems for First Nation adults living on reserves in Canada. Canadian Journal of Public Health, 112(Suppl 1): S20–S28.
Bennett EM, Cramer W, Begossi A, Cundill G, Díaz S, Egoh BN, et al. 2015. Linking biodiversity, ecosystem services, and human well-being: three challenges for designing research for sustainability. Current Opinion in Environmental Sustainability, 14: 76–85.
Berbesque JC, Marlowe FW, Shaw P, and Thompson P. 2014. Hunter-gatherers have less famine than agriculturalists. Biology Letters 10(1): 20130853.
Berkes F, Folke C, and Gadgil M. 1995. Traditional ecological knowledge, biodiversity, resilience, and sustainability. In Biodiversity Conservation: Problems and Policies. Edited by CA Perrings, KG Mäler, C Folke, CS Holling, and BO Jansson. pp. 281–299. Biodiversity conservation: problems and policies. Kluwer Academic Publishers, Dordrecht, The Netherlands.
Berkes F, and Jolly D. 2001. Adapting to Climate Change: Social-Ecological Resilience in a Canadian Western Arctic Community. Conversation Ecology, 5(2): 18.
Bettinger RL, Garvey R, and Tushingham S. 2015. Hunter-Gatherers: Archaeological and Evolutionary Theory. 2nd edition. Springer, New York, New York. 304 p.
Biewener AA. 1989. Scaling Body Support in Mammals: Limb Posture and Muscle Mechanics. Science, 245(4913): 45–48.
Biewener AA. 1990. Biomechanics of Mammalian Terrestrial Locomotion. Science, 250(4984): 1097–1103.
Bilsborough S, and Mann N. 2006. A Review of Issues of Dietary Protein Intake in Humans. International Journal of Sport Nutrition and Exercise Metabolism, 16(2): 129–152.
Binford LR. 1978. Nunamiut: Ethnoarchaeology. Academic Press, New York, New York. 509 p.
Binford LR. 1980. Willow Smoke and Dogs’ Tails: Hunter-Gatherer Settlement Systems and Archaeological Site Formation. American Antiquity, 45(1): 4–20.
Bird DW, Bird RB, and Codding BF. 2009. In pursuit of mobile prey: Martu hunting strategies and archaeofaunal interpretation. American Antiquity, 74(1): 3–29.
Bird DW, Codding BF, Bird RB, Zeanah DW, and Taylor CJ. 2013. Megafauna in a continent of small game: Archaeological implications of Martu Camel hunting in Australia’s Western Desert. Quaternary International, 297: 155–166.
Blackburn TM, Gaston KJ, and Loder N. 1999. Geographic gradients in body size: a clarification of Bergmann's rule. Diversity and distributions, 5(4): 165–174.
Blackenhorn WU. 2000. The evolution of body size: what keeps organisms small? The Quarterly Review of Biology, 75(4): 385–407.
Bonsall MB, and Hassell MP. 2007. Predator-prey interactions. In Theoretical Ecology: Principles and Applications. 3rd ed. Edited by RM May and A McLean. Oxford University Press. pp. 46–61.
Borré K. 1994. The Healing Power of the Seal: The Meaning of Inuit Health Practice and Belief. Arctic Anthropology, 31(1): 1–15.
Bousman CB. 1993. Hunter-Gatherer Adaptations, Economic Risk and Tool Design. Lithic Technology, 18(1-2): 59–86.
Brinkman TJ, Kofinas GP, Chapin III FS, and Person DK. 2007. Influence of Hunter Adaptability on Resilience of Subsistence Hunting Systems. Journal of Ecological Anthropology, 11(1): 58–83.
Broughton JM, Cannon MD, Bayham FE, and Byers DA. 2011. Prey body size and ranking in zooarchaeology: theory, empirical evidence, and applications from the Northern Great Basin. American Antiquity, 76(3): 403–428.
Brown JH, and West GB. 2000. Scaling in Biology. Oxford University Press, New York, New York. 368p.
Bukkens SG. 1997. The nutritional value of edible insects. Ecology of Food and Nutrition, 36(2-4): 287–319.
Burger O, and Ginzburg L. 2009. On size and extinction: a random walk model predicts the body size of lowest risk for mammals. Evolutionary Ecology Research, 11(7): 1017–1029.
Byers DA, and Ugan A. 2005. Should we expect large game specialization in the late Pleistocene? An optimal foraging perspective on early Paleoindian prey choice. Journal of Archaeological Science, 32(11): 1624–1640.
Calder WA. 1996. Size, Function and Life History. Dover Publications, Mineola, New York. 431p.
Canadian Institutes of Health Research, Natural Sciences and Engineering Research Council of Canada, and Social Sciences and Humanities Research Council. 2018. Tri-Council Policy Statement: Ethical Conduct of Research Involving Humans [online]: Available from ethics.gc.ca/eng/policy-politique_tcps2-eptc2_2018.html.
Cardillo M, Mace GM, Jones KE, Bielby J, Bininda-Emonds ORP, Sechrest W, et al. 2005. Multiple Causes of High Extinction Risk in Large Mammal Species. Science, 309(5738): 1239–1241.
Casadevall M, Casinos A, Viladiu C, and Ontanon M. 1990. Scaling of skeletal mass and mineral content in teleosts. Zoologischer Anzeiger, 225(3–4): 144–150.
Chan L, Receveur O, Batal M, David W, Schwartz H, Ing A, et al. 2016. First Nations Food, Nutrition and Environment Study (FNFNES): Results from Alberta (2010). University of Ottawa. 156 p.
Chan L, Receveur O, Batal M, Sadik T, Schwartz H, Ing A, et al. 2018. First Nations Food, Nutrition and Environment Study (FNFNES): Results from Saskatchewan (2010). University of Ottawa. 180 p.
Chan L, Receveur O, Sharp D, Schwartz H, Ing A, and Tikhonov C. 2011. First Nations Food, Nutrition and Environment Study (FNFNES): Results from British Columbia (2008/09). University of Northern British Columbia. 201 p.
Chan L, Receveur O, Sharp D, Schwartz H, Ing A, Fediuk K, et al. 2012. First Nations Food, Nutrition and Environment Study (FNFNES): Results from Manitoba (2010). University of Northern British Columbia. 180 p.
Chan L, Receveur O, Sharp D, Schwartz H, Ing A, Fediuk K, et al. 2014. First Nations Food, Nutrition and Environment Study (FNFNES): Results from Ontario (2011/2012). University of Ottawa. 236 p.
Churchill SE. 1993. Weapon Technology, Prey Size Selection, and Hunting Methods in Modern Hunter-Gatherers: Implications for Hunting in the Palaeolithic and Mesolithic. Archaeological Papers of the American Anthropological Association, 4(1): 11–24.
Clauset A. 2013. How Large Should Whales Be? PLOS ONE, 8(1): e53967.
Clauset A, and Erwin DH. 2008. The Evolution and Distribution of Species Body Size. Science, 321(5887): 399–401.
Codding BF, Whitaker AR, and Bird DW. 2014. Global patterns in the exploitation of shellfish. The Journal of Island and Coastal Archaeology, 9(2): 145–149.
Colding J, and Folke C. 1997. The relations among threatened species, their protection, and taboos. Conservation Ecology, 1(1): 18. [online]: Available from jstor.org/stable/26271644.
Condon RG, Collings P, and Wenzel G. 1995. The best part of life: Subsistence hunting, ethnicity, and economic adaptation among young adult Inuit males. Arctic, 48(1): 31–46.
Cortés E. 2016. Perspectives on the intrinsic rate of population growth. Methods in Ecology and Evolution, 7(10): 1136–1145.
Coulson T, and Godfray HCJ. 2007. Single-species dynamics. In Theoretical Ecology: Principles and Applications. 3rd edition. Edited by R May and A McLean. Oxford University Press. pp. 17–34.
Council of Canadian Academies. 2014. Aboriginal food security in northern Canada: An Assessment of the State of Knowledge. The Expert Panel on the State of Knowledge of Food Security in Northern Canada, Ottawa, ON. 256 p.
Couturier S, Otto RD, Côté SD, Luther G, and Mahoney SP. 2010. Body size variations in caribou ecotypes and relationships with demography. The Journal of Wildlife Management, 74(3): 395–404.
Cummins BD. 1992. Attawapiskat Cree: Land Tenure and Use 1901-1989. Ph.D. thesis, McMaster University, Hamilton, Ontario. 383 p.
Delormier T, Frohlich KL, and Potvin L. 2009. Food and eating as social practice – understanding eating patterns as social phenomena and implications for public health. Sociology of Health & Illness, 31(2): 215–228.
Duhaime F, Chabot M, Fréchette P, Robichaud V, and Proulx S. 2004. The Impact of Dietary Changes Among the Inuit of Nunavik (Canada): A Socioeconomic Assessment of Possible Public Health Recommendations Dealing With Food Contamination. Risk Analysis, 24(4): 1007–1018.
Edgar GJ, and Stuart-Smith RD. 2014. Systematic global assessment of reef fish communities by the Reef Life Survey program. Scientific Data, 1: 140007.
Ernest SKM, Enquist BJ, Brown JH, Charnov EL, Gillooly JF, Savage VM, et al. 2003. Thermodynamic and metabolic effects on the scaling of production and population energy use. Ecology Letters, 6(11): 990–995.
FAO. 1996. Rome declaration on World Food Security [online]: Available from fao.org/docrep/003/w3613e/w3613e00.htm.
Fenchel T. 1974. Intrinsic Rate of Natural Increase: The Relationship with Body Size. Oecologia, 14(4): 317–326.
Firelight and VGFN. 2018. A report on environmental monitoring activities and priorities within the lands of the Vuntut Gwitchin First Nation. [online]: Available from firelight.ca/wp-content/uploads/2016/04/VGFN_Report_FINAL_11MAY2018.pdf.
Ford JD. 2009. Vulnerability of Inuit food systems to food insecurity as a consequence of climate change: a case study from Igloolik, Nunavut. Regional Environmental Change, 9(2): 83–100.
Ford JD, and Pearce T. 2012. Climate change vulnerability and adaptation research focusing on the Inuit subsistence sector in Canada: Directions for future research. The Canadian Geographer, 56(2): 275–287.
Frank KT, Petrie B, Fisher JAD, and Leggett WC. 2011. Transient dynamics of an altered large marine ecosystem. Nature, 477(7362): 86–89.
Froese R, and Pauly D. 2000. FishBase 2000: concepts, design and data sources. ICLARM. 344 p.
Froese R, and Pauly D. 2018. FishBase [online]: Available from fishbase.org.
Fryxell JM, Sinclair ARE, and Caughley G. 2014. Wildlife Ecology, Conservation, and Management. 3rd edition. John Wiley & Sons Ltd, West Sussex, United Kingdom. 524 p.
Garibaldi A, and Turner N. 2004. Cultural Keystone Species: Implications for Ecological Conservation and Restoration. Ecology and Society, 9(3): 1.
Gaston KJ, and Blackburn TM. 1995. Birds, body size and the threat of extinction. Philosophical Transactions of the Royal Society of London, 347(1320): 205–212.
Gregory PJ, Ingram JSI, and Brklacich M. 2005. Climate change and food security. Philosophical Transactions of the Royal Society B, 360(1463): 2139–2148.
Grete HB. 2000. “Sharing”, transfers, transactions and the concept of generalized reciprocity. Senri Ethnological Studies, 53: 193–214.
Grund BS, Surovell TA, and Lyons SK. 2012. Range sizes and the shifts of North American Pleistocene mammals are not consistent with a climatic explanation for extinction. World Archaeology, 44(1): 43–55.
Gurven M, and Hill K. 2009. Why do men hunt? A reevaluation of “man the hunter” and the sexual division of labor. Current Anthropology, 50(1): 51–74.
Guyot M, Dickson C, Paci C, Furgal C, and Chan HM. 2006. Local observations of climate change and impacts on traditional food security in two northern Aboriginal communities. International Journal of Circumpolar Health, 65(5): 403–415.
GRRB. 2021. Research and Management Interests for the GSA. Report prepared for the Gwich’in Reneable Resources Board. [online]: Available from grrb.nt.ca/wp-content/uploads/2021/01/Research-Interests_2018.pdf.
Hames RB, and Vickers WT. 1982. Optimal diet breadth theory as a model to explain variability in Amazonian hunting. The American Ethnological Society, 9(2): 358–378.
Hansen WD, Brinkman TJ, Chapin FS, III, and Brown C. 2013. Meeting Indigenous Subsistence Needs: The Case for Prey Switching in Rural Alaska. Human Dimensions of Wildlife, 18(2): 109–123.
Hawkes K, Hill K, and O’Connell JF. 1982. Why hunters gather: optimal foraging and the Aché of eastern Paraguay. American Ethnologist, 9(2): 379–398.
Hawkes K, O’Connell JF, and Blurton Jones NG. 1991. Hunting income patterns among the Hadza: big game, common goods, foraging goals and the evolution of the human diet. Philosophical Transactions: Biological Sciences, 334(1270): 243–251.
Hayden B. 1972. Population control among hunter/gatherers. World Archaeology, 4(2): 205–221.
Hennemann WW III. 1983. Relationship among body mass, metabolic rate and the intrinsic increase in mammals. Oecologia, 56(1): 104–108.
Hickey G, Pouliot M, Smith-Hall C, Wunder S, and Nielsen MR. 2016. Quantifying the economic contribution of wild food harvests to rural livelihoods: A global-comparative analysis. Food Policy, 62: 122–132.
Inuit Circumpolar Conference (ICC). 1998. Proceedings of the 8th General Assembly Inuit Circumpolar Conference. pp. 68–69.
Imrie DD. 2009. Inuit Knowledge and Adaptation to Sea Ice Change in the Belcher Islands, Nunavut. Ph.D. Thesis. Natural Resources Institute, University of Winnipeg, Winnipeg. 144 p.
James FC. 1970. Geographic size variation in birds and its relationship to climate. Ecology, 51(3): 365–390.
JBNQ NHRC. 1982. The Wealth of the Land: Wildlife Harvests by the James Bay Cree, 1972-73 to 1978-79. James Bay and Northern Québec Native Harvesting Research Committee. 811 p.
JBNQ NHRC. 1988. Final Report: Research to Establish Present Levels of Harvesting for the Inuit of Northern Québec, 1976-1980. James Bay and Northern Québec Native Harvesting Research Committee. 155 p.
Joachim G. 1998. Sources of variability in the reproducibility of food frequency questionnaires. Nutrition and Health, 12: 181–188.
Kaplan H, and Gurven M. 2005. The Natural History of Human Food Sharing and Cooperation: A Review and a New Multi-Individual Approach to the Negotiation of Norms. In Moral sentiments and material interests: The foundations of cooperation in economic life, Vol. 6. Edited by H Gintis, S Bowles, RT Boyd and E Fehr. MIT press. pp. 75–113.
Kelly BC, Ikonomou MG, Blair JD, Morin AE, and Gobas FAPC. 2007. Food Web-Specific Biomagnification of Persistent Organic Pollutants. Science, 317(5835): 236–239.
Kishigami N. 2000. Contemporary Inuit food sharing and hunter support program of Nunavik, Canada. Senri Ethnological Studies, 53: 171–192.
Koster JM. 2008. Hunting with Dogs in Nicaragua: An Optimal Foraging Approach. Current Anthropology, 49(5): 935–944.
Koster JM, Hodgen JJ, Venegas MD, and Copeland TJ. 2010. Is Meat Flavor a Factor in Hunters’ Prey Choice Decisions? Human Nature, 21(3): 219–242.
Kozlowski J, and Gawelczyk AT. 2002. Why are species’ body size distributions usually skewed to the right? Functional Ecology, 16(4): 419–432.
Krause J, Godin JGJ, and Brown D. 1996. Phenotypic variability within and between fish shoals. Ecology, 77(5): 1586–1591.
Kruse J. 2011. Developing an Arctic subsistence observation system. Polar Geography, 34(1-2): 9–35.
Kruse J. Alaska Observation network Subsistence Database [online]: Available from iser.uaa.alaska.edu/Projects/SEARCH-HD/aonsub.htm.
Kuchikura Y. 1988. Efficiency and focus of blowpipe hunting among Semaq Beri Hunter-Gatherers of Peninsular Malaysia. Human Ecology, 16(3): 271–305.
Kuhnlein HV. 1995. Benefits and risks of traditional food for Indigenous Peoples: focus on dietary intakes of Arctic men. Canadian journal of Physiological Pharmacology, 73(6): 765–771.
Kuhnlein HV, and Humphries MM. 2017. Traditional Animal Foods of Indigenous Peoples of Northern North America [online]: Available from traditionalanimalfoods.org/.
Kuhnlein H, and Receveur O. 1996. Dietary change and traditional food systems of Indigenous Peoples. Annual Review of Nutrition, 16(1): 417–442.
Kuhnlein H, and Receveur O. 2007. Local cultural animal food contributes high levels of nutrients for Arctic Canadian indigenous adults and children. Journal of Nutrition, 137(4): 1110–1114.
Kuhnlein H, Receveur O, Soueida R, and Egeland GM. 2004. Arctic Indigenous Peoples Experience the Nutrition Transition with Changing Dietary Patterns and Obesity. Journal of Nutrition, 134(6): 1447–1453.
Kuhnlein H, McDonald M, Spigelski D, Vittrekwa E, and Erasmus B. 2009. Gwich’in traditional food for health: Phase 1. In Indigenous Peoples’ food systems: the many dimensions of culture, diversity and environment for nutrition and health. Edited by H Kuhnlein, B Erasmus and D Spigelski. Food and Agriculture Organization. pp. 45–58.
Lambden J, Receveur O, Kuhnlein HV. 2007. Traditional food attributes must be included in studies of food security in the Canadian Arctic. International Journal of Circumpolar Health, 66(4): 308–319.
Lambden J, Receveur O, Marshall J, and Kuhnlein H. 2006. Traditional and market food access in Arctic Canada is affected by economic factors. International Journal of Circumpolar Health, 65(4): 331–340.
Lawn J, Harvey D, Hill F, and Brulé D. 2002. An Update on Nutrition Surveys in Isolated Northern Communities. A report prepared for Indian and Northern Affairs Canada. 55 p.
Lemire M, Kwan M, Laouan-Sidi AE, Muckle G, Pirkle C, Ayotte P, and Dewailly E. 2015. Local country food sources of methylmercury, selenium and omega-3 fatty acids in Nunavik, Northern Quebec. Science of the Total Environment, 509: 248–259.
Lindstedt SL, Miller BJ, and Buskirk SW. 1986. Home range, time, and body size in mammals. Ecology, 67(2): 413–418.
Lindstrom SG. 1992. Great Basin fisherfolk: Optimal diet breadth modeling the Truckee River aboriginal subsistence fishery. PhD thesis, University of California Davis, Davis, California. 325 p.
Lupo KD. 2006. What Explains the Carcass Field Processing and Transport Decisions of Contemporary Hunter-Gatherers? Measures of Economic Anatomy and Zooarchaeological Skeletal Part Representation. Journal of Archaeological Method and Theory, 13(1): 19–66.
Lupo KD, and Schmitt DN. 2005. Small prey hunting technology and zooarchaeological measures of taxonomic diversity and abundance: Ethnoarchaeological evidence from Central African forest foragers. Journal of Anthropological Archaeology, 24(4): 335–353.
Lupo KD, and Schmitt DN. 2016. When bigger is not better: The economics of hunting megafauna and its implications for Plio-Pleistocene hunter-gatherers. Journal of Anthropological Archaeology, 44: 185–197.
Mackey MGA. 1988. The Impact of Imported Foods on the Traditional Inuit Diet. Arctic Medical Research, 47(1): 128–133.
May RM. 1974. Biological Populations with Nonoverlapping Generations: Stable Points, Stable Cycles, and Chaos. Science, 186(4164): 645–647.
McCay BJ. 1981. Optimal foragers or political actors? Ecological analyses of a New Jersey fishery. American Ethnologist, 8(2): 356–382.
McKinney MA, Peacock E, and Letcher RJ. 2009. Sea ice-associated diet change increases the levels of chlorinated and brominated contaminants in polar bears. Environmental Science and Technology, 43(12): 4334–4339.
Mendonça LET, Vieira WLS, and Alves RRN. 2014. Caatinga Ethnoherpetology: relationships between herpetofauna and people in a semiarid region. Amphibian and Reptile Conserv, 8(1): 24–32.
Morrison NE, Receveur O, Kuhnlein HV, Appavoo DM, Soueida R, and Pierrot P. 1995. Contemporary Sahtu Dene/Métis use of traditional and market food. Ecology of Food and Nutrition, 34: 197–210.
Murphy NJ, Schraer CD, Thiele MC, Boyko EJ, Bulkow LR, Doty BJ, and Lanier AP. 1995. Dietary Change and Obesity Associated with Glucose Intolerance in Alaska Natives. Journal of American Dietetic Association, 95(6): 676–682.
Murray J, and Burt JR. 1991. The Composition of Fish. Tory Advisory Note No. 38, Ministry of Technology, Torry Research Station. 13 p.
Myers JH. 2018. Population cycles: generalities, exceptions, and remaining mysteries. Proceedings of the Royal Society B, 285(1875): 20172841.
Nagaoka L. 2002. Explaining subsistence change in southern New Zealand using foraging theory models. World Archaeology, 34(1): 84–102.
Natcher D, Shirley S, Rodon T, and Southcott C. 2016. Constraints to wildlife harvesting among aboriginal communities in Alaska and Canada. Food Security, 8(6): 1153–1167.
Nelson M, Natcher DC, and Hickey CG. 2005. Social and economic barriers to subsistence harvesting in a northern Alberta aboriginal community. Anthropologica, 47(2): 289–301.
NCP. 2003. Canadian Arctic Contaminants Assessment Report II – Contaminant Levels, Trends and Effects in the Biological Environment. Northern Contaminants Program (NCP), Indian and Northern Affairs Canada, Ottawa. 175 p.
NCP. 2012. Canadian Arctic Contaminants Assessment Report III – Mercury in Canada’s North. Northern Contaminants Program (NCP), Aboriginal Affairs and Northern Development Canada, Ottawa. 276 p.
NCP. 2013. Canadian Arctic Contaminants Assessment Report III – Persistent Organic Pollutants in Canada’s North. Northern Contaminants Program (NCP). Aboriginal Affairs and Northern Development Canada, Ottawa. 487 p.
NERC Centre for Population Biology. 2010. The Global Population Dynamics Database Version 2 [online]: Available from sw.ic.ac.uk/cpb/cpb/gpdd.html.
Nishiwaki M. 1950. On the body weight of whales. The Scientific Reports of the Whales Research Institute. pp. 184–209.
Nunavut Food Security Coalition. 2014. Nunavut Food Security Strategy and Action Plan 2014-16. Nunavut Food Security Coalition. 25 p.
O’Connell JF, Hawkes K, and Jones NB. 1988. Hadza Hunting, Butchering, and Bone Transport and Their Archaeological Implications. Journal of Anthropological Research, 44(2): 113–161.
Olden JD, Hogan ZS, and Zanden MJ. 2007. Small fish, big fish, red fish, blue fish: size-biased extinction risk of the world's freshwater and marine fishes. Global Ecology and Biogeography, 16(6): 694–701.
Outram AK. 2001. Economic anatomy, element abundance and optimality: A new way of examining hunters’ bone transportation choices. In Archaeological Sciences ‘97. Edited by A Millard. Archaeopress. pp. 117–126.
Paci CDJ, Dickson C, Nickels S, Chan L, and Furgal C. 2004. Food Security of Northern Indigenous Peoples in a Time of Uncertainty. In Proceedings of the 3rd Northern Research Forum Open Meeting, Yellowknife, NWT, 15–18 September 2004. pp. 1–11.
Pal S, Haman F, and Robidoux MA. 2013. The costs of local food procurement in two northern Indigenous communities in Canada. Food and Foodways, 21(2): 132–152.
Peres CA. 2000. Effects of Subsistence Hunting on Vertebrate Community Structure in Amazonian Forests. Conservation Biology, 14(1): 240–253.
Peters RH. 1983. The ecological implications of body size. Cambridge University Press, Cambridge, United Kingdom. 329 p.
Peters RH, and Wassenberg K. 1983. The effect of body size on animal abundance. Oecologia, 60(1): 89–96.
Peterson RO, Page RE, and Dodge KM. 1984. Wolves, moose, and the allometry of population cycles. Science, 224(4655): 1350–1352.
Pirkle CM, Muckle G, and Lemire M. 2016. Managing mercury exposure in northern Canadian communities. Canadian Medical Association Journal, 188(14): 1015–1023.
Prange HD, Anderson JF, and Rahn H. 1979. Scaling of Skeletal Mass to Body Mass in Birds and Mammals. The American Naturalist, 113(1): 103–122.
Provencher JF, Gaston AJ, Mallory ML, O’hara PD, and Gilchrist HG. 2010. Ingested plastic in a diving seabird, the thick-billed murre (Uria lomvia), in the eastern Canadian Arctic. Marine Pollution Bulletin, 60(9): 1406–1411.
R Core Team. 2020. R: A language and environment for statistical computing. R Foundation for Statistical Computing, Vienna, Austria. [online]: Available from R-project.org/.
Raia P, Carotenuto F, Passaro F, Fulgione D, and Fortelius M. 2012. Ecological Specialization in Fossil Mammals Explains Cope’s Rule. The American Naturalist, 179(3): 328–337.
Randazzo ML, and Robidoux MA. 2018. The costs of local food procurement in a Northern Canadian First Nation community: an affordable strategy to food security? Journal of Hunter & Environmental Nutrition, 14(5): 662–682.
Rapport DJ, and Turner JE. 1977. Economic Models in Ecology. Science, 195(4276): 367–373. [online]: Available from jstor.org/stable/1743281.
Raymond AW, and Sobel E. 1990. The Use of Tui Chub as Food by Indians of the Western Great Basin. Journal of California and Great Basin Anthropology, 12(1): 2–18.
Richmond CA, and Ross NA. 2009. The determinants of First Nation and Inuit health: A critical population health approach. Health & place, 15(2), 403–411.
Ripple WJ, and Valkenburgh BV. 2010. Linking Top-Down Forces to the Pleistocene Megafaunal Extinctions. Bioscience, 60(7): 516–526.
Robinson JG, and Redford KH. 1986. Intrinsic rate of natural increase in neotropic forest mammals – relationship to phylogeny and diet. Oecologia, 68(4): 516–520.
Roff DA. 1992. The evolution of life histories: theory and analysis. Chapman and Hall, New York, New York. 535 p.
Rogers ES, and Black MB. 1976. Subsistence strategy in the Fish and Hare Period, Northern Ontario: the Weagamow Ojibwa, 1880-1920. Journal of Anthropological Research, 32(1): 1–43.
Rosol R, Powell-Hellyer S, and Chan HM. 2016. Impacts of decline harvest of country food on nutrient intake among Inuit in Arctic Canada: impact of climate change and possible adaptation plan. International Journal of Circumpolar Health, 75(1): 31127.
Ross PS, Ellis GM, Ikonomou MG, Barrett-Lennard LG, and Addison RF. 2000. High PCB concentrations in free-ranging Pacific killer whales, Orcinus orca: effects of age, sex and dietary preference. Marine Pollution Bulletin, 40(6): 504–515.
Royal Commission on Aboriginal Peoples. 1993. Integrated Research Plan: Ethical Guidelines for Research. pp. 37–40. (Appendix B).
Royer MS, and Herrmann TM. 2011. Socioenvironmental changes in two traditional food species of the Cree First Nation of subarctic James Bay. Cahiers de géographie du Québec, 55(156): 575–601.
Samson C, and Pretty J. 2006. Environmental and health benefits of hunting lifestyles and diets for the Innu of Labrador. Food Policy, 31(6): 528–553.
Schipper J, Chanson JS, Chiozza F, Cox NA, Hoffman M, Katariya V, et al. 2008. The Status of the World’s Land and Marine Mammals: Diversity, Threat, and Knowledge. Science, 322(5899): 225–230.
Schmitz OJ, and Lavigne DM. 1984. Intrinsic rate of increase, body size, and specific metabolic rate in marine mammals. Oecologia, 62(3): 305–309.
de Schutter O. 2010. Report submitted by the Special Rapporteur on the right to food, Olivier De Schutter. Presented to the Humans Rights Council, United Nations. 21 p.
Sebens KP. 1987. The Ecology of Indeterminate Growth in Animals. Annual Review of Ecology and Systematics, 18:371–407.
Simms SR. 1987. Behavioral Ecology and Hunter Gatherer Foraging: An Example from the Great Basin. BAR International Series No. 381, British Archaeological Reports, Oxford, United Kingdom. 157 p.
Sirén AH, and Wilkie DS. 2016. The effects of ammunition price on subsistence hunting in an Amazonian village. Oryx, 50(1): 47–55.
Smith EA. 1991. Inujjuamiut Foraging Strategies: Evolutionary Ecology of an Arctic Hunting Economy. Aldine de Gruyter, New York, New York. 455 p.
Smith EA, Bettinger RL, Bishop CA, Blundell V, Cashdan E, Casimir MJ, et al. 1983. Anthropological Applications of Optimal Foraging Theory: A Critical Review. Current Anthropology, 24(5): 625–651.
Speakman JR. 2005. Review: Body size, energy metabolism and lifespan. The Journal of Experimental Biology, 208(9): 1717–1730.
Speth JD. 1989. Early hominid hunting and scavenging: the role of meat as an energy source. Journal of Human Evolution, 18(4): 329–343.
Speth JD, and Spielmann KA. 1983. Energy Source, Protein Metabolism, and hunter-Gatherer Subsistence Strategies. Journal of Anthropological Archaeology, 2(1): 1–31.
Stearns SC. 1992. The evolution of life histories. 1st edition. Oxford University Press, Oxford, United Kingdom. 262 p.
Stephens DW, and Krebs JR. 1986. Foraging theory. Princeton University Press, Princeton, New Jersey. 262 p.
Stephens PA, Vieira MV, Willis SG, and Carbone C. 2019. The limits to population density in birds and mammals. Ecology Letters, 22(4): 654–663.
Surovell TA, and Waguespack NM. 2009. Human Prey Choice in the Late Pleistocene and Its Relations to Megafaunal Extinctions. In American Megafaunal Extinctions at the End of the Pleistocene. Edited by G Haynes. Springer. pp. 77–105.
Thompson SD. 1987. Body size, duration of parental care, and the intrinsic rate of natural increase in eutherian and metatherian mammals. Oecologia, 71(2): 201.
Thompson JC, Carvalho S, Marean CW, and Alemseged Z. 2019. Origins of the Human Predatory Pattern. Current Anthropology, 60(1): 1–23.
Thorson T. 1961. The Partitioning of Body Water in Osteichthyes: Phylogenetic and Ecological Implications in Aquatic Vertebrates. Biological Bulletin, 120(2): 238–254.
Turner NJ, Łuczaj ŁJ, Migliorini P, Pieroni A, Dreon AL, Sacchetti LE, and Paoletti MG. 2011. Edible and tended wild plants, traditional ecological knowledge and agroecology. Critical Reviews in Plant Sciences, 30(1-2): 198–225.
Ugan A. 2005. Does size matter? Body size, mass collection, and their implications for understanding prehistoric foraging behaviour. American Antiquity, 70(1): 75–89.
UNEP. 2019. Global Mercury Assessment – 2018. United Nations Environment Programme (UNEP), Chemicals and Health Branch. pp. 60.
Usher PJ. 1971. The Bankslanders: Economy and Ecology of a Frontier Trapping Community: Volume 2. Economy and Ecology, Department of Indian Affairs and Northern Development, Ottawa. 169 p.
Usher PJ. 2002. Inuvialuit Use of the Beaufort Sea and its Resources 1960-2000. Arctic, 55(1): 18–28.
Usher PJ, and Wenzel G. 1987. Native Harvest Surveys and Statistics: A Critique of Their Construction and Use. Arctic, 40(2): 145–160.
Vuntut Gwitchin First Nation, and Smith S. 2009. People of the lakes: stories of our Van Tat Gwich’in elders = googwandak nakhwach’ànjòo Van Tat Gwich’in. University of Alberta Press, Edmonton, Canada. 456 p.
Waguespack NM, and Surovell TA. 2003. Clovis hunting strategies, or how to make out on plentiful resources. American Antiquity, 68(2): 333–352.
Walch A, Bersamin A, Loring P, Johnson R, and Tholl M. 2018. A scoping review of traditional food security in Alaska. International Journal of Circumpolar Health, 77: 1419678.
Wein EE, and Freeman MMR. 1995. Frequency of traditional food use by three Yukon First Nations living in four communities. Arctic Institute of North America, 48(2): 161–171.
Weinbaum KZ, Brashares JS, Golden CD, and Getz WM. 2013. Searching for sustainability: are assessments of wildlife harvests behind the times? Ecology Letters, 16(1): 99–111.
Wenzel GW. 2009. Canadian Inuit subsistence and ecological instability – if the climate changes, must the Inuit? Polar Research 28: 89–99.
Wesche SD, and Chan HM. 2010. Adapting to the Impacts of Climate Change on Food Security among Inuit in the Western Canadian Arctic. EcoHealth, 7(3): 361–373.
West G. 2017. Scale: the universal laws of growth, innovation, sustainability, and the pace of life in organisms, cities, economies, and companies. Penguin Random House, New York, New York. 479 p.
Wheatley B, and Paradis S. 1995. Exposure of Canadian Aboriginal peoples to methylmercury. Water, Air, and Soil Pollution, 80(1): 3–11.
White EP, Ernest SKM, Kerkhoff AJ, and Enquist BJ. 2007. Relationships between body size and abundance in ecology. Trends in Ecology & Evolution, 22(6): 323–330.
Wilman H, Belmaker J, Simpson J, de la Rosa C, Rivadeneira MM, and Jetz W. 2014. EltonTraits 1.0: Species-level foraging attributes of the world's birds and mammals. Ecology, 95(7): 2027.
Winterhalder BP. 1981. Foraging strategies in the boreal forest: an analysis of Cree hunting and gathering. In Hunter-Gatherer Foraging Strategies: Ethnographic and Archeological Analyses. Edited by B Winterhalder and EA Smith. University of Chicago Press. pp. 66–98.
Winterhalder BP. 1986. Optimal foraging: simulation studies of diet choice in a stochastic environment. Journal of Ethnobiology, 6(1): 205–223.
Winterhalder BP. 1987. The Analysis of Hunter-Gatherer Diets: Stalking an Optimal Foraging Model. In Food and Evolution: Toward a Theory of Human Food Habits. Edited by M Harris and EB Ross. Temple University Press. pp. 635.
Winterhalder BP, and Lu F. 1997. A Forager-Resource Population Ecology Model and Implications for Indigenous Conservation. Conservation Biology, 11(6): 1354–1364.
Yesner DR. 1989. Moose Hunters of the Boreal Forest? A Re-examination of Subsistence Patterns in the Western Subarctic. Arctic, 42(2): 97–108.
Supplementary Materials
Supplementary Material 1 (DOCX / 14 KB)
- Download
- 14.09 KB
Supplementary Material 2 (DOCX / 13.6 KB)
- Download
- 13.61 KB
Supplementary Material 3 (DOCX / 120 KB)
- Download
- 120.73 KB
Supplementary Material 4 (DOCX / 1.10 MB)
- Download
- 1.10 MB
Supplementary Material 5 (DOCX / 583 KB)
- Download
- 583.33 KB
Supplementary Material 6 (DOCX / 113 KB)
- Download
- 113.08 KB
Supplementary Material 7 (DOCX / 156 KB)
- Download
- 156.34 KB
Supplementary Material 8 (DOCX / 21.2 KB)
- Download
- 21.22 KB
Information & Authors
Information
Published In
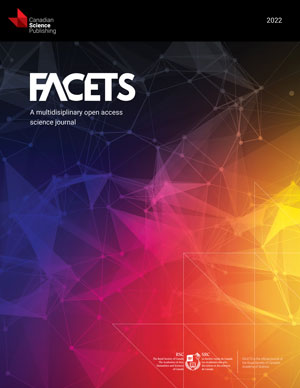
FACETS
Volume 7 • Number 1 • January 2022
Pages: 286 - 318
Editor: Stephen B. Heard
History
Received: 3 April 2020
Accepted: 30 September 2021
Version of record online: 3 March 2022
Copyright
© 2022 Brammer et al. This work is licensed under a Creative Commons Attribution 4.0 International License (CC BY 4.0), which permits unrestricted use, distribution, and reproduction in any medium, provided the original author(s) and source are credited.
Data Availability Statement
All relevant data are within the paper and in the Supplementary Material.
Key Words
Sections
Subjects
Plain Language Summary
How species body size is important for traditional food systems based on wild fish and animals
Authors
Author Contributions
JRB, AKM, LSC, XG-B, ML-C, M-LL, EKS, and MMH conceived and designed the study.
JRB, AKM, LSC, XG-B, ML-C, M-LL, EKS, and MMH performed the experiments/collected the data.
JRB, AKM, LSC, XG-B, ML-C, M-LL, EKS, and MMH analyzed and interpreted the data.
JRB, AKM, XG-B, ML-C, M-LL, MNN, EKS, and MMH contributed resources.
JRB, AKM, XG-B, ML-C, M-LL, MNN, EKS, and MMH drafted or revised the manuscript.
Competing Interests
The authors have declared that no competing interests exist.
Metrics & Citations
Metrics
Other Metrics
Citations
Cite As
Jeremy R. Brammer, Allyson K. Menzies, Laurence S. Carter, Xavier Giroux-Bougard, Manuelle Landry-Cuerrier, Melanie-Louise Leblanc, Mikhaela N. Neelin, Emily K. Studd, and Murray M. Humphries. 2022. Weighing the importance of animal body size in traditional food systems. FACETS.
7: 286-318.
https://doi.org/10.1139/facets-2020-0023
Export Citations
If you have the appropriate software installed, you can download article citation data to the citation manager of your choice. Simply select your manager software from the list below and click Download.
Cited by
1. Humanity’s diverse predatory niche and its ecological consequences
2. The Evolution of Paleolithic Hunting Weapons: A Response to Declining Prey Size