The need for speed
Abstract
This paper reviews the challenges posed by the physics of the interaction of high-peak power femtosecond lasers with ultrathin foil targets. Initially designed to produce warm solid-density plasmas through the isochoric heating of solid matter, the interaction of an ultrashort pulse with ultrathin foils is becoming more and more complex as the laser intensity is increased. The dream of achieving very hot solid density matter with extreme specific energy density faces several bottlenecks discussed here as related to the laser technology, to the complexity of the physical processes, and to the limits of our current time-resolved instrumentations.
Introduction
A few years after the invention of the laser, high-temperature plasmas were produced by focusing a high-power laser pulse, in the tens of picosecond range, on the surface of a solid target (Basov and Krokhin 1964), and neutron emission was observed, associated with thermonuclear DD reactions (Basov et al. 1968a). Various successive experiments and modeling extracted the basic physics regulating this ablative laser–matter interaction in which thermal conduction can dominate on a short time scale before the hydrodynamic separation (Basov et al. 1968b; Kidder 1968; Bobin et al. 1969; Floux et al. 1970). At the end of the 1960s at the Lawrence Livermore National Laboratory, Nuckolls et al. (1972) realized that a powerful nanosecond laser beam could be used to compress matter at very high density. The implosion-scheme based on ablatively driven compression induced by nanosecond laser pulses started the field of Inertial Confinement Fusion (ICF). The challenge of ICF is to control the target compression induced either directly by a symmetric laser beam irradiation or indirectly by a cloud of X-rays generated inside a Hohlraum. The plasma parameters (density, temperature, shock waves, ionization states, opacities) and the associated hydrodynamics (and related instabilities), which are crucial properties to control and optimize the fusion yield, are changing rapidly in both space and time during the laser–matter interaction. This quest for ICF has been, since then, driving the development of very high-energy lasers with durations in the nanosecond regime and energies in the MJ range.
The invention of the laser Chirped Pulse Amplification (CPA) technique by Strickland and Mourou (1985) allowed for stacking energy in a very short time inferior to the picosecond. This interaction experiment between such a high-energy short pulse and a solid target indicated that it was possible to keep the plasma gradient scale-length very short during the heating, thus bringing matter at solid density to high temperatures on a time scale shorter than dynamics (Falcone et al. 1986; Murnane and Falcone 1988; Kieffer et al. 1989; Rosen 1990). A physical regime of isochoric heating, close to early models, was becoming accessible with the CPA laser technology.
With the CPA technique, the laser power has been propelled over the past 20 years to the Petawatt–Exawatt range with very high energies (in the 100 J to kJ range) delivered in ultrashort pulse duration (in tens of femtosecond) opening the door to relativistic physics at extreme intensity and particle acceleration among other directions (Mourou et al. 1998; Danson et al. 2019). This progress has exacerbated the need for speed. Henri Pépin (Fig. 1) at Institut National de la Recherche scientifique (INRS) was one of the first to capture the potential of the CPA technique for the applications of laser–matter interaction.
Fig. 1.

Several Canadian groups at the University of Alberta, University of British Columbia, National Research Council, INRS, University of Toronto, Université de Sherbrooke, McGill University, and Université Laval played significant roles in the exploration of these new territories of laser–matter interaction. This paper reviews some achievements in the domain of hot solid-density plasmas.
The isochoric heating concept
Isochoric heating is a very old concept initially explored by Guillaume Amontons in 1699 and Joseph Louis Gay-Lussac in 1802. Robert Stirling built the first engine introducing isochoric heating in the hydrodynamic cycle in 1816. An isochoric process is a thermodynamic process in which the volume of a closed system remains constant. This process is part of the combustion engine cycle and it is traditionally associated with the heating or cooling of a gas inside a container that cannot be deformed. The heat transfer into the closed system changes only the internal energy of the system.
With a nonconstrained system, as a slab of solid material, isochoric heating at large temperature can be realized only if the heat deposition is realized in a pulsed way on a time scale shorter than the matter expansion dynamics. This requires a pulsed heating source with a very short duration. The interaction of a CPA high-energy laser pulse with an ultrathin foil solid target, with a thickness much smaller than the thermal penetration depth (defined as the depth reached by the thermal conduction front inside a thick solid target at the end of the heating laser pulse, and which is typically in the few hundreds of nanometers range) and equal to or smaller than the skin depth (defined as the depth at which the laser electromagnetic field penetrates in a solid target, which is in the ten nanometer range), emerged as a way to produce hot solid-density plasmas slabs, through isochoric heating, with high temperature and homogeneous temperature distribution (Kieffer et al. 1994; Saemann et al. 1999). The heating phase at solid density is then followed by a fast-adiabatic decompression and cooling of the plasma slab (Falcone et al. 1986).
The challenge of laser-based isochoric heating of solids to high temperatures is achieving a specific energy density in the 104–106 MJ/kg range, or pressure in the few to hundreds Giga-Pascal range, during the high-power laser–matter interaction inside an irradiated target, accelerating the matter to velocities in the 105–106 m/s range. Such hot and dense plasmas have been proposed as sources of ultrashort bright X-ray pulses (Falcone et al. 1986; Kieffer et al. 1993a), test benches for the determination of equation of state (Foord et al. 2004), and for the study of warm dense plasmas (Forsman et al. 1998). Isochoric heating of matter at solid density can be realized by direct laser heating producing thin plasma slabs (Kieffer et al. 1994), discussed in the present review, or by protons (Patel et al. 2003) or X-rays to generate a volumetric heating (Dyer et al. 2003; Hoidn and Seidler 2018).
The need for speed
Since the first experiment reported in 1834 by Charles Wheatstone (1834) on the measurement of the velocity of electricity with a revolving mirror, and since the 1864 proposal from Ducos-du-Hauron to create an apparatus to record single-shot pictures with short exposure times and taking a series of pictures at various rates (Ducos du Hauron 1864), the challenge of speed in measurement techniques remains amazingly modern and actual for ultrashort laser-produced plasmas. The field of high-speed photography made impressive progress with many technologies presented in several reviews (Courtney-Pratt 1957; Coleman 1963; Shapiro 1977; Korobkin et al. 1978; Fanchenko 1999; Schelev 2000; Ray 2002). However, this field needs to be extended to short wavelength with ultimate temporal resolutions.
The size of the irradiated matter or the duration of the interaction impose a minimum time scale of interest and thus a required temporal resolution. To see detailed information in μm size target submitted to these extreme conditions, it is therefore necessary to have sub-picosecond time resolutions. With shorter laser pulses and multi-Petawatt (PW) systems now available, the constraint on time resolution is now set by the duration of the interaction process and resolution in the 10–100 femtosecond (fs) would be extremely useful.
X-ray emission spectroscopy is one of the key diagnostics to understand the interaction physics and the various nonequilibrium plasmas processes taking place during the interaction (Agarwal 1991; Griem 1997). Time-resolved X-ray emission spectroscopy remains particular since the physicist needs a tool capable either to record a monochromatic image with an extremely short exposure time or to measure a time-resolved spectrum with the highest spectral and fastest temporal resolutions with a large dynamic range.
Much progress has been realized on laser-based light sources with higher and higher power, shorter and shorter pulse durations, down to the attosecond territory for sub-keV X-ray source (Corkum 1993), and on autocorrelation techniques able to probe fs durations. However, the resolution of the ultrafast X-ray emission spectroscopy of ultrashort laser-generated plasma remains limited to around 300–500 fs.
The hot plasma slab
If a very thin foil, with a thickness well below the thermal penetration length, is irradiated by a very short pulse having a steep rise time, then the laser–plasma interaction can take place in a gradient scale-length well shorter than the laser wavelength, and energy deposition can be realized at near-solid density (Kieffer et al. 1989). Electrons are thus heated extremely rapidly and the ultrathin foil is uniformly heated before any hydrodynamic expansion occurs. At low temperature, the electron–ion equilibration time can be shorter than the pulse duration and the system reach the equilibrium state during the laser pulse.
Two experimental methods have been currently explored to produce such hot plasma slabs from ultrathin foil solids irradiated by laser. The first one is based on the use of a judicious choice of laser intensity and pulse duration (a few hundreds of fs) to generate a radiation pressure confinement effect limiting hot plasma expansion during the laser pulse (Peyrusse et al. 1995; Gallant et al. 2000a). However, this method remains limited to a very narrow range of parameters and is very sensitive to beam quality and 2-D/3-D effects. A second method relies on the use of a sufficiently short pulse (few tens of fs) to heat up the target instantaneously at near-solid density on a time scale shorter than the hydrodynamic expansion time. Figs. 2 and 3 schematically show the difference between the ablative regime obtained with a thick target and a long (nanosecond range) pulse (Fig. 2) and the isochoric heating regime achieved with a short pulse (fs range) and a thin foil (Fig. 3).
Fig. 2.

Fig. 3.

Experiments realized with ultrathin foils, fs laser pulses, and with low to intermediate laser intensities demonstrated the possibility to generate high-density (near-solid density) plasmas with temperatures in the range from a few eV to a few hundred eV and allowed the measure of plasma conductivity at solid or near-solid density as a function of the temperature and at low excitation specific energy densities between 20 MJ/kg (Ao et al. 2006; Chen et al. 2018) and 103 MJ/kg (Mo et al. 2017; Mahieu et al. 2018).
As an example, we consider here a recent experiment done at the Advanced Laser Light Source by University of Alberta and University of British Columbia groups (Mo et al. 2017). A 50 nm Al foil is heated at 25 eV at solid density (generating a warm dense plasma) with a 30 fs pulse focused at 1015 W/cm2. The absorption is measured to be around 45%. From the irradiated volume and the experimentally measured absorption, we can deduce a specific energy density of 103 MJ/kg (Mo et al. 2017). Using a very simple model for energy deposition at near-solid density (Kieffer et al. 1989), we obtain for the absorption A = 2.7 × 108 νef Z (1/(λμ1/3 τ2/3 TK13/6)), and for the temperaturewhere TK is the electron temperature in K, I0 is the incident laser intensity in W/m2, τ is the pulse duration in s, d0 is the target thickness in m, Z is the average ionization, and λμ is the laser wavelength in μm. The parameter νef is defined as the coefficient of the collision frequency v = νef Z ne/T3/2. Assuming that the average ionization at this intensity is Z = 6, and taking νef = 2.6 × 10−8 (m3 K3/2/s), one obtains A = 53% and T = 30 eV, which agrees with the experimental data. The specific energy density is thus, for Al, ε (MJ/kg) = 12 τ4/11 (I0 τ/d0)6/11. In principle, with ultrathin foils (10–200 nm thickness range) the radiation Planck mean free path is larger than the initial foil thickness d0, and the radiation contribution to the specific energy density remains negligible.
Measuring the values of the plasma slab parameters (temperature, specific energy density, electron density) reached through the laser energy deposition is an unbelievable challenge since space and time scales are very short and the system can be out of equilibrium. One idea to access initial specific energy density of heated ultrathin foils is to measure the adiabatic cooling of the foil, looking to a plasma density decompression time τ (ne = n0 e−t/τ where ne is the electron density at the time t and n0 is the initial plasma density), which should be faster when the temperature is higher. The initial specific energy density could thus be obtained from the expansion history ε = cs2/γ = d02/γτ2, where cs is the ion acoustic velocity and γ is the ratio of specific heats. The slab electron density time history is inferred from time resolved X-ray spectroscopy of plasma line emissions.
The temporal resolution challenges for X-rays
Streak camera coupled to a spectrometer became progressively the most adapted tool to see the dynamics of various ionization states in laser-produced hot plasmas. Modern streak cameras are based on the tube converter concept introduced in 1949 by J.S. Courtney Pratt (Courtney-Pratt 1949) and refined by Russian groups in the mid-1950s (Butslov et al. 1959). The laboratories involved in ICF research started to develop their own high-speed instrumentation in the 1960s and cameras with resolutions in the 50–100 ps range were available at the beginning of the 1970s (Huston 1964; Zavoisky and Fanchenko 1965; Bradley 1973). The first X-ray streak camera was developed at the Lawrence Livermore National Laboratory (LLNL) in 1974 with a 15 ps time resolution (McConaghy and Coleman 1974). At the same time, the Commissariat aux Energies Alternatives (CEA) groups introduced tube with lamellar electron optics that have been progressively refined to achieved 2 ps temporal resolution (Boutry et al. 1979, 1983; Girard et al. 1984; Sauneuf 1989).
In 1989, the Berkeley group (Murnane et al. 1989) demonstrated that it was possible to achieve a 2 ps time resolution with a commercial Kentech X-ray streak camera modified by using a high extraction voltage, a photocathode delivering very narrow distribution of photoelectrons and fast sweeping. Similar time resolution was demonstrated with the C850X X-ray streak camera designed by CEA and based on the bi-lamellar electron optics concept (Mens et al. 1991). Fig. 4 shows the architecture of the bi-lamellar tube.
Fig. 4.

The 2 ps limit was essentially due to transit time dispersion between the photocathode and the acceleration plane. At the beginning of the 1990s, several groups started to develop new X-ray streak tubes to push the time resolution limits in the sub-picosecond range. By applying a pulse field of 270 kV/cm to the photocathode accelerating structure, the LLNL group (Shepherd et al. 1995) was the first to break the 1 ps barrier achieving in 1995 a 900 fs temporal resolution. Similar temporal resolution (880 fs) was achieved in 1996 with meander-type deflection plates (Chang et al. 1996). The INRS group achieved similar 850 fs time resolution in 1996 with the PX1 sub-picosecond X-ray streak camera (Coté et al. 1997a), specifically developed for time-resolved X-ray spectroscopy, based on a shorter bilamellar tube and minimizing the dispersion of photoelectron transit time inside the tube (in a near paraxial mode) and focusing aberrations. LLNL achieved later, in 2000, a 500 fs time resolution with the TREX camera (Shepherd et al. 2004). Several generations of sub-picosecond X-ray streak cameras (PX, HX, FX, and FXR cameras based on different streak tube designs) were developed at INRS between 1995 and 2005. However, the record 350 fs temporal resolution in single-shot mode in the keV range was obtained with the PX1 camera in 2000 with a cathode extraction field of 250 kV, while maintaining a high spatial resolution of 40 μm along an axis perpendicular to the time dispersion direction (Gallant et al. 2000b).
Time-resolved X-ray emission spectroscopy
The first significant step in time-resolved X-ray spectroscopy was realized in 1976 by LLNL (Atwood et al. 1976) with a 15 ps time resolution (McConaghy and Coleman 1974), the spectral information was obtained with a set of K-edge filters in front of the streak camera’s slit. The first time-resolved spectrum, published in 1980, was obtained with a streak camera having a 70 ps time resolution coupled to a crystal spectrometer (Key et al. 1980). Line emission from a neon-filled micro-balloon irradiated by a 100 ps laser pulse was observed giving access to the time history of the expanding plasma over 500 ps (Kauffman et al. 1982). Very quickly after these first results, several experiments reported various time-resolved spectra, with time resolution in the 10–100 ps range, deriving the temporal dependence of the electron temperature (Burnett et al. 1984; Gizzi et al. 1994) and showing the local heating of hot electron population (Burnett et al. 1984).
Various improvements have been done in parallel on photocathodes (Henke et al. 1981) enabling shorter time resolution at the beginning of the 1980s and, as an example, implosions times of irradiated micro-balloons were measured with an absolutely timed X-ray streak camera and a 15 ps resolution (Letzring et al. 1983). Streak tubes for fusion have been progressively refined and improved (Jaanimagi et al. 1988; Kalantar et al. 1997; Kimbrough et al. 2001; Donaldson et al. 2002; Kuba et al. 2004; Marley et al. 2012; Opachich et al. 2012; Howorth et al. 2016; Zuber et al. 2016) and applied to time-resolved spectroscopy of large plasmas with high dynamic range. Time and two-dimensional space-resolved monochromatic imaging, obtained with an X-ray streak camera coupled to a Johann spectrometer, has been realized and was used to study the dynamics of Ne-like population in collisionally excited X-ray laser (Nantel et al. 1993).
The first time-resolved spectra (from Aluminum He-resonance line to Be-like short-lived emission) with a 2 ps time resolution was obtained in 1993 (Kieffer et al. 1993b) and showed the importance of non-Maxwellian character of the electron distribution (as previously demonstrated with X-ray polarization spectroscopy (Kieffer et al. 1992)) and demonstrated the power of ultrafast X-ray spectroscopy technique to study ultrashort laser–matter interaction (Tsakiris 1990; Gizzi et al. 1994; Kieffer et al. 1996; Coté et al. 1997b; Bastiani-Ceccotti et al. 2004; Nilson et al. 2015). In 2000, two experiments (Gallant et al. 2000b; Shepherd et al. 2004) reported measurements of time-resolved K-shell line emissions with a 500 fs resolution with the TREX camera (Shepherd et al. 2003, 2004) and 350 fs resolution with the PX1 camera (Gallant et al. 1997, 2000b). Since then, to the best of our knowledge, no other time-resolved keV X-ray spectroscopic measurements have been reported with faster resolution, and these two experiments remain the fastest X-ray spectroscopy ever realized with resolution in the 400–500 fs range.
To measure the initial hot solid-density plasma slab, several experiments have been realized to time-resolved Li-like lines emitted by a hot highly ionized plasma that provides the information on the electron density. The result of our experiments with the PX1 camera (Gallant et al. 2000b) at an intensity of 5 × 1018 W/cm2, 0.53 μm wavelength, and short pulses (150 fs) showed (Fig. 5) that a 50 nm Al foil decompress extremely fast when the interaction is purely thermal, with no hot electrons (less than 1% of the laser energy in the hot electron distribution).
Fig. 5.

The Al Heα resonance line (1s2-1s2p) at 7.76 Å shows a very fast peak, with a full width at half maximum (FWHM) of 700 fs, followed by a tail which is a few picoseconds long. One-dimensional simulations (Gallant et al. 2000a) in these experimental conditions give a 300 fs plasma slab decompression time, a 600 fs Heα FWHM, and an initial electron temperature around 1 keV at near-solid density, corresponding to a specific energy density of around 5 × 104 MJ/kg. This measured Heα resonance line FWHM (700 fs) is, to my best knowledge, the shortest duration ever measured for such a highly ionized emission.
The presence of a hot electron population (10% and more of the laser energy in the hot electron distribution), generated by nonlinear effects during the interaction between a p-polarized pulse and the foil, changes completely the foil dynamics. At the same laser intensity, but in p polarization, the same wavelength and same experimental geometry (Gallant et al. 2000a), the decompression rate is longer (3 ps) and much slower than expected (0.6 ps) with 1-D simulations and observed when no hot electrons are present (Fig. 5). The specific energy densities that could be deduced from the measured decompression time in our experiments with p-polarized pulse is lower and less than 104 MJ/kg.
The dynamic range challenge
In the ultrafast domain, the X-ray streak camera temporal resolution was improved with the INRS PX1 camera but to the detriment of the dynamic range. The dynamic range is a fundamental parameter for laser–matter interaction experiments, which is related to the electronic tube throughput (Jaanimagi et al. 1995; Mens et al. 1997). In the sub-picosecond regime, it can be severely limited by the specific conception of the tube. To increase the time resolution, the electron trajectories can be selected inside the tube and only paraxial trajectories can be used to achieve temporal information. In these conditions the tube transmission and signal to noise ratio remain low, and rapid photocathode depletion, space charge effects, and saturation effects can limit the dynamic range of the instrument to less than a factor 10 at the best temporal resolution (Edwards et al. 2007). Several groups started to use streak cameras coupled to laser-driven photoconductive switches and demonstrated the ultrafast jitter-free time-resolved signal (Margulis et al. 1980; Mourou and Knox 1980; Knox and Mourou 1981; Yen et al. 1984; Maksimchuk et al. 1996; Coté et al. 1998; Chernousov et al. 2000; Belzile et al. 2002; Eagleton and James 2003; Liu et al. 2003; Sun et al. 2005; Bonté et al. 2007; Feng et al. 2007; Martel et al. 2007). Sub-picosecond resolution (600–800 fs) with no limitation on the dynamic range was obtained in 1998 with the PX1 streak camera by jitter-free accumulation of time-resolved signal (Coté et al. 1998) with high repetition rate lasers. In this accumulation mode, the ultimate time resolution of the detection system is the convolution of the intrinsic streak camera resolution with the trigger precision. The FX1 camera, constructed by INRS group in 2002 and specifically designed to operate in signal averaging (accumulation) mode, was coupled to a conical crystal spectrometer and was used for sub-picosecond spectroscopy of laser irradiated Ar cluster X-ray source (Bonté et al. 2007). Very long-term stability (a few hours) of this device has been demonstrated allowing time resolved spectroscopy of low brightness X-ray sources (Bonté et al. 2007).
The ultrashort laser–matter interaction challenge at extreme intensity
When the laser intensity is increased to try to achieve solid density plasmas with higher excitation specific energy densities and temperatures, the interaction between the laser pulse and an ultrathin foil is no longer purely thermal and becomes more and more complex and new processes and mechanisms have been predicted. In the ultrathin target (thickness in the nanometer range) the direct laser field may play an important role in the electron dynamics and in the foil expansion since the skin depth is in the 10 nm range (Steinke et al. 2010). Hot electron populations can also change the foil dynamics through resistive heating, hot electron recirculation, and magnetic fields (Fuchs et al. 1999; MacKinnon et al. 2002). This has been widely studied both experimentally and theoretically for laser intensities in the 1018–1022 W/cm2 range with ultrathin foils and also in the context of ion acceleration with foils having thickness in the micrometer range. Ultrathin foil could also be directly accelerated by the laser radiation pressure as a piston (Esirkepov et al. 2004; Macchi et al. 2009; Schlegel et al. 2009; Albright et al. 2010; Yan et al. 2010) by high Mach number collisionless shocks (Forslund and Shonk 1970; Silva et al. 2004) or by the radiation transport, which could become dominant over thermal conduction (Sigel et al. 1992; Ditmire et al. 1996). At a high intensity, time-resolved optical probing of ultrathin foil shows a very fast expansion of the target with rear side foil velocity along the target normal (axial) reaching up be 8 × 108 cm/s 10 ps after the main pulse with 10 and 30 nm thin foil and decreasing with time (Fourmaux et al. 2011). High axial expansion velocities (2.3 × 108 cm/s at early expansion times) decreasing with time have already been reported in one experiment at high intensity (2 × 1018 W/cm2) with long pulses (1 ps) and a thick target (Beg et al. 1997). The observation of such a large foil velocity, related to high pressure, is intriguing. Special conditions are achieved in this regime, which could involve strong return currents, recirculating hot electrons and radiation effect, and magnetic field among other effects. Some scenarios also involve Coulomb explosion, a well-known process occurring with molecules and clusters, and it also happens in plasmas during the interaction of a relativistic pulse with an ultrathin foil target (Fourkal et al. 2005; Mourou et al. 2006; Guskov 2020) related to the response of the matter to the hot electron population leaving the target and inducing strong charge separation effects in a thin foil. Radial return currents composed of a high electron density population at low temperature cannot compensate for the hot electron current going through the target or leaving the target.
In such a regime, the Coulomb field can eventually overcome the ambipolar field, which sends back hot electrons towards the target. The coulomb field is given by Eel = (1/6)πkc Zenid0, where kc =8.987 × 109 Nm2/C2 is the Coulomb constant and ni is the ion density uncompensated by electrons inside the target. With ni = 3 × 1022 cm−3 (which corresponds to 50% of the initial ion density ni0) d0 = 30 nm, and Z = 10, the kinetic energy (equal to the electrostatic energy) of a proton accelerated by the Coulomb explosion is 2 MeV, which is similar to the experimental observation (2.5 MeV) (Fourmaux et al. 2011). The electrostatic effect is, in these conditions, accelerating heavier ions at a velocity cs = 7 × 106 m/s, which is similar to the foil relaxation velocities observed in Fourmaux et al. (2011). The pressure per unit mass inside the target in those conditions is P/ρ = dcs/τL = 7 × 106 MJ/kg, which indicates that the system could be put in motion by the electrostatic pressure giving an effect similar to a very high specific energy. More experiments are needed in this regime to capture the complexity of this interaction regime.
Perspectives
Several new concepts have been proposed during the past 20 years to go beyond the few hundred fs temporal resolution (Fanchenko and Schelev 1999), but practical implementation on time-resolved X-ray spectroscopy has not been realized. A combination of an electrostatic field and a radio-frequency (RF) field electron mirror has been proposed to compensate for electron trajectories and reduce temporal resolution to 10 fs (Tron and Gorlov 2007). Electron mirrors and double cylindrical system have been proposed (Jaanimagi 2004) to control the electron time dispersion across a streak tube, but space charge effects (Siwick et al. 2002) remain a concern and such systems have not been incorporated into a streak camera. A THz camera has also been developed and incorporates a gas as a source of photoelectrons. The ionization is localized in an external THz field that is streaking the photoelectron energy spectrum. Resolution of around 10 fs (Frühling et al. 2009; Gorgisyan et al. 2015) has been obtained with free electron laser (FEL) sub-keV X-ray pulses, but such a streaking technique is difficult to implement to simultaneously resolve an X-ray pulse spectrally and temporally. The use of noble gas atoms as a photocathode has also achieved temporal resolution in the sub-picosecond with extreme ultraviolet (XUV) pulses (Warntjes et al. 2001). An electron pulse-dilation technique in which an electronic signal is slowed down using velocity dispersion through a vacuum drift tube has also been explored (Cai et al. 2016; Hares et al. 2016) and used with some success, but time resolution remains still large.
In conclusion, despite all this progress, time-resolved X-ray emission spectroscopy and time-resolved X-ray absorption spectroscopy (Shepherd et al. 2001; Lecherbourg et al. 2006) with extreme (10 fs range) time resolution remains elusive.
Acknowledgements
I would like to thank Henri Pépin who welcomed me in Quebec more than 40 years ago, opening the door to a long friendship, and who invited me to share with him this fascinating adventure with ultrafast laser–matter interaction physics. This article is dedicated to him for his 80th birthday. All this work was made possible thanks to the fantastic invention of the Chirped Pulse Amplification technique, for which D. Strickland and G. Mourou shared the 2018 Nobel Prize. I express all my gratitude to both for their particular attention to my work and unfailing support and guiding over the years. I would like also to thank all the students, researchers and collaborators who have been involved in this fs time-resolved spectroscopy field with me over the years at one time or another, C. Belzile, N. Burnett, M. Chaker, C.Y. Coté, F. Dorchies, P. Forget, S. Fourmaux, J. Fuchs, P. Jaanimagi, T.W. Johnston, P. Gallant, D. Gonthier, C. Goulmy, L. Lecherbourg, C. Martel, F. Martin, J.P. Matte, A. Mens, J.-L. Miquel, M. Nantel, O. Peyrusse, V. Pitre, F. Poitras, F. Salin, M. Sutton, J.C. Rebuffie; Thanks also to D. Villeneuve who taught me so many things from the plasma to the molecule. I would like to thank P. Corkum for his extraordinary inspiration and guiding which allowed me to create the Advanced Laser Light Source (ALLS) laboratory at INRS in 2002. This work is supported by NSERC, FCI, the Canada Research Chair program, FQRNT, INRS, MEI.
References
Agarwal BK. 1991. X-ray spectroscopy. 2nd edition. Springer Verlag.
Albright BJ, Yin L, Hegelich BM, Bowers KJ, Huang C, Henig A, et al. 2010. Ultraintense laser interaction with nanoscale targets: a simple model for layer expansion and ion acceleration. Journal of Physics: Conference Series, 244: 042022.
Ao T, Ping Y, Widmann K, Price DF, Lee E, Tam H, et al. 2006. Optical properties in nonequilibrium phase transitions. Physical Review Letters, 96: 055001.
Atwood DT, Coleman LW, Larsen JT, and Storm EK. 1976. Time-resolved X-ray spectral studies of laser-compressed targets. Physical Review Letters, 37: 499–502.
Basov NG, and Krokhin ON. 1964. Conditions for heating up of a plasma by the radiation from an optical generator. Soviet Physics JETP, 19: 123–125.
Basov NG, Kriukov P, Zakharov S, Senatsky Y, and Tchekalin S. 1968a. Experiments on the observation of neutron emission at a focus of high-power laser radiation on a lithium deuteride surface. IEEE Journal of Quantum Electronics, 4(11): 864–867.
Basov NG, Gribkov VA, Krokiun ON, and Sklizkov GV. 1968b. High temperature effects of intense laser emission focused on a solid target. Soviet Physics JETP, 27(4): 575–582.
Bastiani-Ceccotti S, Audebert P, Nagels-Silvert V, Geindre JP, Gauthier JC, Adam JC, et al. 2004. Time-resolved analysis of the X-ray emission of femtosecond-laser-produced plasmas in the 1.5-keV range. Applied Physics B, 78: 905–909.
Beg FN, Bell AR, Dangor AE, Danson CN, Fews AP, Glinsky ME, et al. 1997. A study of picosecond laser-solid interactions up to 1019 W cm−2. Physics of Plasmas, 4: 447–457.
Belzile C, Kieffer JC, Cote CY, Oksenhendler T, and Kaplan D. 2002. Jitter-free subpicosecond streak cameras (invited). Review of Scientific Instruments, 73: 1617–1620.
Bobin JL, Delobeau F, De Giovanni G, Fauquignon C, and Floux F. 1969. Temperature in laser-created deuterium plasmas. Nuclear Fusion, 9: 115–120.
Bonté C, Harmand M, Dorchies F, Magnan S, Pitre V, Kieffer JC, et al. 2007. High dynamic range streak camera for subpicosecond time-resolved X-ray spectroscopy. Review of Scientific Instruments, 78: 043503.
Boutry B, Delmare C, and Fleurot N. 1979. Picosecond streak camera with a lamellar optics image converter tube. In SPIE Proceedings. Vol. 189, pp. 507–512.
Boutry B, Cavailler C, and Fleurot N. 1983. P 600/650 X-ray streak camera with optimized spatio-temporal resolution. In SPIE Proceedings. Vol. 348, pp. 766–772.
Bradley DJ. 1973. Electron optical image tubes and image tube streak cameras. US Patent 3,761,614.
Burnett NH, Enright GD, Avery A, Loen A, and Kieffer JC. 1984. Time-resolved Kα spectra in high-intensity laser-target interaction. Physical Review A, 29: 2294–2297.
Butslov MM, Zavoiskii EK, Plahov AG, Smolkin GE, and Fanchenko SD. 1959. Image tube camera. In Proceedings of the 4th International Congress on High Speed Photography. pp. 230–242.
Cai H, Zhao X, Liu J, Xie W, Bai Y, Lei Y, et al. 2016. Dilation framing camera with 4 ps resolution. APL Photonics, 1: 016101.
Chang Z, Rundquist A, Zhou J, Murnane MM, Kapteyn HC, Liu X, et al. 1996. Demonstration of a sub-picosecond X-ray streak camera. Applied Physics Letters, 69: 133–135.
Chen Z, Mo M, Soulard L, Recoules V, Hering P, Tsui YY, et al. 2018. Interatomic potential in the nonequilibrium warm dense matter regime. Physical Review Letters, 121: 075002.
Chernousov YD, Guidi V, Logachov PV, and Tecchio L. 2000. Proposal for a deflecting system for streak camera with sub-ps resolution and wide dynamic range. Nuclear Instruments and Methods in Physics Research Section A: Accelerators, Spectrometers, Detectors and Associated Equipment, 451: 541–544.
Coleman KR. 1963. Ultra-high-speed photography. Reports on Progress in Physics, 26: 269–305.
Corkum PB. 1993. Plasma perspective on strong field multiphoton ionization. Physical Review Letters, 71(13): 1994–1997.
Coté CY, Kieffer J-C, Gallant P, Rebuffie J-C, Goulmy C, Maksimchuk AM, et al. 1997a. Development of a subpicosecond large-dynamic-range X-ray streak camera. In 22nd International Congress on High Speed Photography and Photonics (1996), SPIE Proceedings. Vol. 2869, pp. 956–964.
Coté CY, Kieffer JC, and Peyrusse O. 1997b. Picosecond time-resolved spectroscopy of a controlled preformed plasma heated by an intense subpicosecond laser pulse. Physical Review E, 56: 992–1000.
Coté CY, Kaplan D, Bouvier M, Eidmann K, Tesar J, and Kieffer JC. 1998. A laser-triggered ultrafast streak camera for the measurement of ultrashort events on the femtosecond time scale. In SPIE Proceedings. Vol. 3414, pp. 280–287.
Courtney-Pratt JS. 1949. A new photographic method for studying fast transient phenomena (discussion on detonation). Proceedings of the Royal Society of London. Series A, Mathematical and Physical Sciences, 204(1076): 27–29 (22 November 1950) and Research, 2: 287–294.
Courtney-Pratt JS. 1957. A review of the methods of high-speed photography. Reports on Progress in Physics, 20: 379–432.
Danson CN, Haefner C, Bromage J, Butcher T, Chanteloup J-CF, Chowdhury EA, et al. 2019. Petawatt and exawatt class lasers worldwide. High Power Laser Science and Engineering, 7: E54.
Ditmire T, Gumbrell ET, Smith RA, Mountford L, and Hutchinson MH. 1996. Supersonic ionization wave driven by radiation transport in a short-pulse laser-produced plasma. Physical Review Letters, 77: 498–501.
Donaldson WR, Boni R, Keck RL, and Jaanimagi PA. 2002. A self-calibrating, multichannel streak camera for inertial confinement fusion applications. Review of Scientific Instruments, 73: 2606–2615.
Ducos du Hauron L. 1er mars 1864. Appareil destiné à reproduire photographiquement une scène quelconque avec toutes les transformations qu’elle a subies pendant un temps déterminé. Brevet français de 15 ans, sous le n° 61976.
Dyer G, Sheppherd R, Kuba J, Fill E, Wootton A, Patel P, et al. 2003. Isochoric heating of solid aluminium with picosecond X-ray pulses. Journal of Modern Optics, 50: 2495–2505.
Eagleton RT, and James SF. 2003. Dynamic range measurements on streak image tubes with internal and external microchannel plate image amplification. Review of Scientific Instruments, 74: 2215–2219.
Edwards MH, Booth N, Zhai Z, Tallents GJ, Dzelzainis T, Lewis CLS, et al. 2007. Space charge effects in the axis-photonique PX-1 X-ray streak camera. In SPIE Proceedings. Vol. 6703, 67030L.
Esirkepov T, Borghesi M, Bulanov SV, Mourou G, and Tajima T. 2004. Highly efficient relativistic-ion generation in the laser-piston regime. Physical Review Letters, 92: 175003.
Falcone RW, and Murnane M 1986. Proposal for a femtosecond X-ray light source. In Short wavelength coherent radiation. Vol. 147. Edited by DT Atwood and J Bokor. AIP, New York, New York. pp. 81–85.
Fanchenko SD. 1999. Femtosecond photography lessons. In SPIE Proceedings. Vol. 3516, pp. 15–35.
Fanchenko SD, and Schelev MY. 1999. Down to the fundamental lower limit of direct time cognition. In SPIE Proceedings. Vol. 3516, pp. 916–924.
Feng J, Shin HJ, Nasiatka JR, Wan W, Young AT, Huang G, et al. 2007. An X-ray streak camera with high spatio-temporal resolution. Applied Physics Letters, 91: 134102.
Floux F, Cognard D, Denoeud L-G, Piar G, Parisot D, Bobin JL, et al. 1970. Nuclear fusion reactions in solid-deuterium laser-produced plasma. Physical Review A, 1(3): 821–824.
Foord ME, Reisman DB, and Springer PT. 2004. Determining the equation-of-state isentrope in an isochoric heated plasma. Review of Scientific Instruments, 75: 2586–2589.
Forslund D, and Shonk CR. 1970. Formation and structure of electrostatic collisionless shocks. Physical Review Letters, 25: 1699–1702.
Forsman A, Ng A, Chiu G, and More RM. 1998. Interaction of femtosecond laser pulses with ultrathin foils. Physical Review E, 58: R1248–R1251.
Fourkal E, Velchev I, and Ma C-M. 2005. Coulomb explosion effect and the maximum energy of protons accelerated by high-power lasers. Physical Review E, 71: 036412.
Fourmaux S, Buffechoux S, Gnedyuk S, Albertazzi B, Capelli D, Lecherbourg L, et al. 2011. Laser-based proton acceleration on ultrathin foil with a 100-TW-class high intensity laser system. In SPIE Proceedings. Vol. 8007, 80070L.
Frühling U, Wieland M, Gensch M, Gebert T, Schütte B, Krikunova M, et al. 2009. Single-shot terahertz-field-driven X-ray streak camera. Nature Photonics, 3: 523–528.
Fuchs J, Adam JC, Blanchot N, Gallant P, Gremillet L, Héron A, et al. 1999. Experimental study of laser penetration in overdense plasmas at relativistic intensities. II: explosion of thin foils by laser driven fast electrons. Physics of Plasmas, 6: 2569–2578.
Gallant P, Jiang Z, Fuchs J, Kieffer J-C, Pepin H, Gontier D, et al. 1997. Subpicosecond time-resolved X-ray spectroscopy of plasmas produced by high-intensity ultrashort laser pulses. In SPIE Proceedings. Vol. 3157, pp. 44–51.
Gallant P, Jiang Z, Chien CY, Forget P, Dorchies F, Kieffer JC, et al. 2000a. Spectroscopy of solid density plasmas generated by irradiation of thin foils by a fs laser. Journal of Quantitative Spectroscopy and Radiative Transfer, 65: 243–252.
Gallant P, Forget P, Dorchies F, Jiang Z, and Kieffer JC. 2000b. Characterization of a subpicosecond X-ray streak camera for ultrashort laser-produced plasmas experiments. Review of Scientific Instruments, 71: 3627–3633.
Girard A, Loty C, Roux JP, Noel J, Rebuffie JC, and Allamargot JL. 1984. P 700: a new high speed streak tube with lamellar electron optics. In SPIE Proceedings. Vol. 491, pp. 58–66.
Gizzi LA, Giulietti D, Giulietti A, Afshar-Rad T, Biancalana V, Chessa P, et al. 1994. Characterization of laser plasmas for interaction studies. Physical Review E, 49: 5628–5643.
Gorgisyan I, Juranic PN, Ischebeck R, Stepanov A, Schlott V, Pradervand C, et al. 2015. The new design of the THz streak camera at PSI. In SPIE Proceedings. Vol. 9512, 95120D.
Griem H. 1997. Principles of plasma spectroscopy. Cambridge University Press.
Guskov SY. 2020. Amplification of separated electric charge field due to the capture of laser-produced fast electrons oscillating near thin target. Physics of Plasmas, 27: 122109.
Hares JD, Dymoke-Bradshaw AKL, Hilsabeck TJ, Kilkenny JD, Morris D, Horsfield CJ, et al. 2016. A demonstration of ultra-high time resolution with a pulse-dilation photo-multiplier. Journal of Physics: Conference Series, 717: 012093.
Henke BL, Knauer JP, and Premaratne K. 1981. The characterization of X-ray photocathodes in the 0.1–10-keV photon energy region. Journal of Applied Physics, 52: 1509–1520.
Hoidn O, and Seidler GT. 2018. Nonlocal heat transport and improved target design for X-ray heating studies at X-ray free electron lasers. Physical Review B, 97: 035145.
Howorth JR, Milnes JS, Fisher Y, Jadwin A, Boni R, and Jaanimagi PA. 2016. The development of an improved streak tube for fusion diagnostics. Review of Scientific Instruments, 87: 11D447.
Huston AE. 1964. An image tube camera for photography of plasmas. Applied Optics, 3: 1231–1234.
Jaanimagi PA. 2004. Breaking the 100-fs barrier with a streak camera. In SPIE Proceedings. Vol. 5194, pp. 171–183.
Jaanimagi PA, Bradley DK, Duff J, Gregory GG, and Richardson MC. 1988. Time-resolving X-ray diagnostics for ICF (invited). Review of Scientific Instruments, 59: 1854–1859.
Jaanimagi PA, Mens A, and Rebuffie J-C. 1995. Photoelectron throughput in streak tubes. In SPIE Proceedings. Vol. 2549.
Kalantar DH, Bell PM, Costa RL, Hammel BA, Landen OL, Orzechowski TJ, et al. 1997. Characterization of X-ray streak cameras for use on Nova. In 22nd International Congress on High Speed Photography and Photonics (1996), SPIE Proceedings. Vol. 956.
Kauffman RL, Matthews DL, Kilkenny JD, and Lee RW. 1982. Time-resolved X-ray line diagnostics of laser-produced plasmas. LLNL Report UCRL-87422.
Key MH, Lewis CLS, Lunney JG, Moore A, Ward JM, and Thareja RK. 1980. Time-resolved X-ray spectroscopy of laser produced plasmas. Physical Review Letters, 44: 1669–1672.
Kidder R. 1968. Application of lasers to the production of high-temperature and high-pressure plasma. Nuclear Fusion, 8: 3–12.
Kieffer JC, Audebert P, Chaker M, Matte JP, Pépin H, Johnston TW, et al. 1989. Short-pulse laser absorption in very steep plasma density gradients. Physical Review Letters, 62: 760–763.
Kieffer JC, Matte JP, Pépin H, Chaker M, Beaudoin Y, Johnston TW, et al. 1992. Electron distribution anisotropy in laser-produced plasmas from X-ray line polarization measurements. Physical Review Letters, 68: 480–483.
Kieffer JC, Chaker M, Matte JP, Pépin H, Côté CY, Beaudoin Y, et al. 1993a. Ultrafast X-ray sources. Physics of Fluids B: Plasma Physics, 5: 2676–2681.
Kieffer JC, Chaker M, Côté CY, Beaudoin Y, Pépin H, Chien CY, et al. 1993b. Time-resolved kiloelectron-volt spectroscopy of ultrashort plasmas. Applied Optics, 32: 4247–4252.
Kieffer JC, Côté CY, Jiang Z, Beaudoin Y, Chaker M, and Peyrusse O. 1994. Towards hot solid-density plasmas with ultra-high-intensity sub-picosecond lasers. Canadian Journal of Physics, 72: 802–807.
Kieffer JC, Jiang Z, Ikhlef A, Cote CY, and Peyrusse O. 1996. Picosecond dynamics of a hot solid-density plasma. Journal of the Optical Society of America B, 13: 132–137.
Kimbrough JR, Bell PM, Christianson GB, Lee FD, Kalantar DH, Perry TS, et al. 2001. National Ignition Facility core X-ray streak camera. Review of Scientific Instruments, 72: 748–750.
Knox W, and Mourou G. 1981. A simple jitter-free picosecond streak camera. Optics Communications, 37: 203–206.
Korobkin VV, Stepanov BM, Fanchenko SD, and Schelev MY. 1978. Pico-femtosecond electron-optical photography. Optical and Quantum Electronics, 10: 367–381.
Kuba J, Shepherd R, Booth R, Stewart RE, Lee ECW, Audebert P, et al. 2004. Subpicosecond streak camera measurements at LLNL: from IR to X-rays. In SPIE Proceedings. Vol. 5194, pp. 183–192.
Lecherbourg L, Fourmaux S, Kieffer JC, Martin F, Pépin H, Chaker M, et al. 2006. Source X-UV pour la spectroscopie d’absorption en régime femtoseconde. Journal de Physique IV France, 138: 63–72.
Letzring SA, Thorsos EI, Friedman WD, Seka W, and Rizzo JE. 1983. An absolutely timed X-ray streak camera for laser fusion experiments. Journal of Applied Physics, 54: 6302–6306.
Liu J, Wang J, Shan B, Wang C, and Chang Z. 2003. An accumulative X-ray streak camera with sub-600-fs temporal resolution and 50-fs timing jitter. Applied Physics Letters, 82: 3553–3555.
Macchi A, Veghini S, and Pegoraro F. 2009. “Light sail” acceleration reexamined. Physical Review Letters, 103: 085003.
MacKinnon AJ, Sentoku Y, Patel PK, Price DW, Hatchett S, Key MH, et al. 2002. Enhancement of proton acceleration by hot-electron recirculation in thin foils irradiated by ultraintense laser pulses. Physical Review Letters, 88: 215006.
Mahieu B, Jourdain N, Ta Phuoc K, Dorchies F, Goddet JP, Lifschitz A, et al. 2018. Probing warm dense matter using femtosecond X-ray absorption spectroscopy with a laser-produced betatron source. Nature Communications, 9: 3276.
Maksimchuk A, Kim M, Workman J, Korn G, Squier J, Du D, et al. 1996. Signal averaging X-ray streak camera with picosecond jitter. Review of Scientific Instruments, 67: 697–699.
Margulis W, Sibbett W Jr, Taylor JR, and Bradley DJ. 1980. Reduction of jitter in streak-camera synchronization with picosecond laser pulses. Optics Communications, 32: 331–333.
Marley EV, Shepherd R, Fulkerson S, James L, Emig J, and Norman D. 2012. Ultra fast X-ray streak camera for ten inch manipulator based platforms. Review of Scientific Instruments, 83: 10E106.
Martel C, Fourmaux S, Côté CY, Magnan S, Lecherbourg L, and Kieffer JC. 2007. Development of streak cameras for time-resolved experiments at the advanced laser light source laboratory. In SPIE Proceedings. Vol. 6279, 627909.
McConaghy CF, and Coleman LW. 1974. Picosecond X-ray streak camera. Applied Physics Letters, 25: 268–270.
Mens A, Dalmasso JM, Sauneuf R, Verrecchia R, Roth JA, Tomasini F, et al. 1991. C 850X picosecond high-resolution streak camera. In SPIE Proceedings. Vol. 1358, pp. 315–324.
Mens A, Adolf A, Gontier D, Goulmy C, Jaanimagi PA, Quine C, et al. 1997. Photoelectron current saturation in streak tubes used for high-dynamic-range measurements on inertial confinement fusion lasers. In 22nd International Congress on High Speed Photography and Photonics (1996), SPIE Proceedings. Vol. 2869, pp. 139–148.
Mo MZ, Chen Z, Fourmaux S, Saraf A, Kerr S, Otani K, et al. 2017. Measurements of ionization states in warm dense aluminum with betatron radiation. Physical Review E, 95: 053208.
Mourou G, and Knox W. 1980. A picosecond jitter streak camera. Applied Physics Letters, 36: 623–626.
Mourou GA, Barty CPJ, and Perry MD. 1998. Ultrahigh-intensity lasers: physics of the extreme on a tabletop. Physics Today, 51(1): 22–28.
Mourou GA, Tajima T, and Bulanov SV. 2006. Optics in the relativistic regime. Review of Modern Physics, 78: 309–371.
Murnane MM, and Falcone RW. 1988. Short pulse laser interaction with solids. In SPIE Proceedings. Vol. 913, pp. 5–14.
Murnane MM, Kapteyn HC, and Falcone RW. 1989. High density plasmas produced by ultrafast laser pulses. Physical Review Letters, 62: 155–158.
Nantel M, Kieffer JC, La Fontaine B, Pépin H, Enright GD, Villeneuve DM, et al. 1993. Dynamics of Ne-like populations in the germanium X-ray laser. Physics of Fluids B: Plasma Physics, 5: 4465–4472.
Nilson PM, Solodov AA, Davies JR, Theobald W, Mileham C, Stoeckl C, et al. 2015. Time-resolved Kα spectroscopy measurements of hot-electron equilibration dynamics in thin-foil solid targets: collisional and collective effects. Journal of Physics B: Atomic, Molecular and Optical Physics, 48: 224001.
Nuckolls J, Wood L, Thiessen A, and Zimmerman G. 1972. Laser compression of matter to super-high densities: thermonuclear (CTR) applications. Nature, 239: 139–142.
Opachich YP, Palmer N, Homoelle D, Hatch B, Bell P, Bradley D, et al. 2012. X-ray streak camera cathode development and timing accuracy of the 4ω ultraviolet fiducial system at the National Ignition Facility. Review of Scientific Instruments, 83: 10E123.
Patel PK, Mackinnon AJ, Key MH, Cowan TE, Foord ME, Allen M, et al. 2003. Isochoric heating of solid-density matter with an ultrafast proton beam. Physical Review Letters, 91: 125004.
Pépin H, Dick K, Martineau J, and Parbhakar K. 1972. Plasma produced by irradiation of thin foils of aluminum by a CO2-TEA laser. Physics Letters A, 38: 203–204.
Pépin H, Martin F, Grek B, Johnston TW, Kieffer JC, and Mitchel G. 1979. Evidence of nonlinear processes from X-ray spectra of CO2 laser-irradiated targets. Journal of Applied Physics, 50: 6784–6788.
Pépin H, Alaterre P, Chaker M, Fabbro R, Faral B, Toubhans I, et al. 1987. X-ray sources for microlithography created by laser radiation at λ = 0.26 μm. Journal of Vacuum Science & Technology B: Microelectronics Processing and Phenomena, 5: 27–32.
Pépin H, Comtois D, Vidal F, Chien CY, Desparois A, Johnston TW, et al. 2001. Triggering and guiding high voltage large-scale leader discharges with sub-Joule ultrashort laser pulse. Physics of Plasmas, 8: 2532–2539.
Peyrusse O, Busquet M, Kieffer JC, Jiang Z, and Côté CY. 1995. Generation of hot solid-density plasmas by laser radiation pressure confinement. Physical Review Letters, 75: 3862–3865.
Ray SF (Editor). 2002. High speed photography and photonics. SPIE Press.
Rosen M. 1990. Scaling laws for femtosecond laser-plasma interaction. In SPIE Proceedings. Vol. 1229, pp. 160–167.
Saemann A, Eidmann K, Golovkin IE, Mancini RC, Andersson E, Förster E, et al. 1999. Isochoric heating of solid aluminum by ultrashort laser pulses focused on a tamped target. Physical Review Letters, 82: 4843–4846.
Sauneuf R. 1989. Time-resolved detectors applied to laser-produced plasmas. In SPIE Proceedings. Vol. 1140, pp. 466–474.
Schelev MY. 2000. Femtosecond photoelectronics—past, present, and future. Physics-Uspekhi, 43: 931–946.
Schlegel T, Naumova N, Tikhonchuk VT, Labaune C, Sokolov IV, and Mourou G. 2009. Relativistic laser piston model: ponderomotive ion acceleration in dense plasmas using ultraintense laser pulses. Physics of Plasmas, 16: 083103.
Shapiro SL (Editor). 1977. Ultrashort light pulses. Vol. 18, 1st edition. Topics in Applied Physics. Springer-Verlag, New York, New York.
Shepherd R, Booth R, Price D, Bowers M, Swan D, Bonlie J, et al. 1995. Ultrafast X-ray streak camera for use in ultrashort laser-produced plasma research. Review of Scientific Instruments, 66: 719–721.
Shepherd R, Audebert P, Chenais-Popovics C, Geindre JP, Fajardo M, Iglesias C, et al. 2001. Absorption of bound states in hot, dense matter. Journal of Quantitative Spectroscopy and Radiative Transfer, 71: 711–719.
Shepherd R, Audebert P, Chen H-K, Fournier KB, Peyreusse O, Moon S, et al. 2003. Satellite and opacity effects on resonance line shapes produced from short-pulse laser heated foils. Journal of Quantitative Spectroscopy and Radiative Transfer, 81: 431–440.
Shepherd R, Audebert P, Booth R, Young B, Bonlie J, Nelson D, et al. 2004. Time resolved spectroscopy of ultrashort pulse laser generated X rays using von Hámos crystal spectroscopy. Review of Scientific Instruments, 75: 3765–3767.
Sigel R, Tsakiris GD, Lavarenne F, Massen J, Fedosejevs R, Eidmann K, et al. 1992. Experimental investigation of radiation heat waves driven by laser-induced Planck radiation. Physical Review A, 45: 3987–3996.
Silva LO, Marti M, Davies JR, Fonseca RA, Ren C, Tsung FS, et al. 2004. Proton shock acceleration in laser-plasma interactions. Physical Review Letters, 92: 015002.
Siwick BJ, Dwyer JR, Jordan RE, and Miller RJD. 2002. Ultrafast electron optics: propagation dynamics of femtosecond electron packets. Journal of Applied Physics, 92: 1643–1648.
Steinke S, Henig A, Schnürer M, Sokollik T, Nickles P, Jung D, et al. 2010. Efficient ion acceleration by collective laser-driven electron dynamics with ultra-thin foil targets. Laser and Particle Beams, 28: 215–221.
Strickland D, and Mourou G. 1985. Compression of amplified chirped optical pulses. Optics Communications, 56(3): 219–221.
Sun K-X, Nishimura W, Perry T, and Compton S. 2005. A second-generation X-ray streak camera with true large format, high dynamic range, and high reliability. In SPIE Proceedings. Vol. 5920, 592008.
Tron AM, and Gorlov T. 2007. New type photocathode for X-ray streak camera of the 10-fs resolution. In Proceedings of DIPAC. TUPC 18, pp. 183–185.
Tsakiris GD. 1990. Applications of a soft X-ray streak camera in laser-plasma interaction studies. In SPIE Proceedings. Vol. 1358, pp. 174–181.
Warntjes JBM, Gürtler A, Osterwalder A, Rosca-Pruna F, Vrakking MJJ, and Noordam LD. 2001. Atomic streak camera operating in the extreme ultraviolet. Review of Scientific Instruments, 72: 3205–3207.
Wheatstone C. 1834. An account of some experiments to measure the velocity of electricity and the duration of electric light. Philosophical Transactions of the Royal Society of London, 124: 583–591.
Yan XQ, Tajima T, Hegelich M, Yin L, and Habs D. 2010. Theory of laser ion acceleration from a foil target of nanometer thickness. Applied Physics B, 98: 711–721.
Yen R, Downey PM, Shank CV, and Auston DH. 1984. Low jitter streak camera triggered by subpicosecond laser pulses. Applied Physics Letters, 44: 718–720.
Zavoisky EK, and Fanchenko SD. 1965. Image converter high-speed photography with 10−9–10−14 sec time resolution. Applied Optics, 4: 1155–1167.
Zuber C, Bazzoli S, Brunel P, Burillo M, Fronty JP, Gontier D, et al. 2016. Performance of laser Megajoule’s X-ray streak camera. Review of Scientific Instruments, 87: 11E303.
Information & Authors
Information
Published In
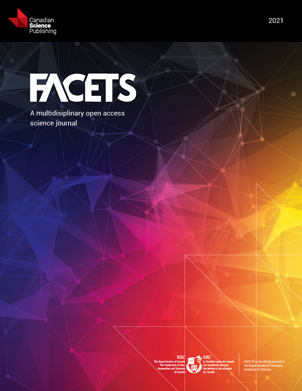
FACETS
Volume 6 • Number 1 • January 2021
Pages: 1390 - 1408
Editor: Vincent Tabard-Cossa
History
Received: 5 February 2021
Accepted: 12 April 2021
Version of record online: 19 August 2021
Copyright
© 2021 Kieffer. This work is licensed under a Creative Commons Attribution 4.0 International License (CC BY 4.0), which permits unrestricted use, distribution, and reproduction in any medium, provided the original author(s) and source are credited.
Data Availability Statement
All relevant data are within the paper.
Key Words
Sections
Subjects
Authors
Author Contributions
All conceived and designed the study.
All performed the experiments/collected the data.
All analyzed and interpreted the data.
All contributed resources.
All drafted or revised the manuscript.
Competing Interests
The author has declared that no competing interests exist.
Metrics & Citations
Metrics
Other Metrics
Citations
Cite As
Jean-Claude Kieffer. 2021. The need for speed. FACETS.
6: 1390-1408.
https://doi.org/10.1139/facets-2021-0011
Export Citations
If you have the appropriate software installed, you can download article citation data to the citation manager of your choice. Simply select your manager software from the list below and click Download.
Cited by
1. Excitation signatures of isochorically heated electrons in solids at finite wave number explored from first principles
2. Laser induced damage threshold and incubation effects of high-power laser system optics