From coast to coast to coast: ecology and management of seagrass ecosystems across Canada
Abstract
Seagrass meadows are among the most productive and diverse marine ecosystems, providing essential structure, functions, and services. They are also among the most impacted by human activities and in urgent need of better management and protection. In Canada, eelgrass (Zostera marina) meadows are found along the Atlantic, Pacific, and Arctic coasts, and thus occur across a wide range of biogeographic conditions. Here, we synthesize knowledge of eelgrass ecosystems across Canada’s coasts, highlighting commonalities and differences in environmental conditions, plant, habitat, and community structure, as well as current trends and human impacts. Across regions, eelgrass life history, phenology, and general species assemblages are similar. However, distinct regional differences occur in environmental conditions, particularly with water temperature and nutrient availability. There is considerable variation in the types and strengths of human activities among regions. The impacts of coastal development are prevalent in all regions, while other impacts are of concern for specific regions, e.g., nutrient loading in the Atlantic and impacts from the logging industry in the Pacific. In addition, climate change represents a growing threat to eelgrass meadows. We review current management and conservation efforts and discuss the implications of observed differences from coast to coast to coast.
1. Introduction
Seagrasses are marine flowering plants that form structurally complex and highly productive habitats in coastal areas worldwide (Hemminga and Duarte 2008). Seagrass meadows provide valuable ecosystem functions and services, including sediment stabilization, carbon storage, and critical habitat for a range of marine species (Barbier et al. 2011; Duffy et al. 2015); therefore, they are a focus for protection and management around the world (DFO 2009a; Marion and Orth 2010; Unsworth et al. 2019). Despite their ecological and economic significance, seagrass meadows face numerous threats associated with coastal development, pollution, aquaculture, invasive species, and fisheries practices (Orth et al. 2006; Murphy et al. 2019). Additionally, seagrass meadows are influenced by the direct and indirect effects of climate change including rising temperatures, sediment erosion, and acidification (Marbà and Duarte 2010; Koch et al. 2013; Wilson and Lotze 2019). These pressures have resulted in global losses of seagrass meadows over past decades and centuries (Lotze et al. 2006; Orth et al. 2006; Waycott et al. 2009), with 14% of all seagrass species considered at risk of extinction (Short et al. 2011). Fortunately, increasing conservation efforts have been successful in slowing or even reversing declining trends in some regions (de los Santos et al. 2019). The extent to which global trends reflect the status of seagrass in Canada is unclear. There were no Canadian sites included in the global quantitative review by Waycott et al. (2009), and the positive trajectories reported by de los Santos et al. (2019) pertained solely to Europe. The vast range of conditions, from temperate to polar coasts, and historical and current pressures under which seagrass habitat may be found in Canada suggest the potential for extensive intrajurisdictional variation in seagrass status.
In Canada, the Department of Fisheries and Oceans (DFO) has designated eelgrass (Zostera marina) as an Ecologically Significant Species (ESS) and eelgrass habitat has been prioritized for conservation and inclusion in future marine protected areas (DFO 2007, 2009a, 2019; Gale et al. 2018). However, except for some local management of coastal habitats, such as by First Nations or community groups, and general efforts to manage human impacts on marine ecosystems, direct management of eelgrass at provincial or federal levels is limited. With the longest coastline in the world (202 080 km), Canada has vast jurisdiction over temperate to polar coastal habitats. Yet, despite eelgrass meadows being a major habitat type in nine of Canada’s 12 marine biogeographic regions (hereafter referred to as bioregions, DFO 2009b), to date there has been no published national eelgrass assessment of its status or distribution and eelgrass in Canada is inadequately mapped (McKenzie et al. 2020). This provides a window of opportunity to inform future research and management by synthesizing current knowledge of eelgrass, its ecological value, and threats across Canada’s three coasts.
Canada is home to six seagrass species (Zostera marina, Z. japonica, Ruppia maritima, Phyllospadix scouleri, P. serrulatus, and P. torreyi) of which eelgrass (Z. marina) is the most widely distributed and abundant species found in soft-bottom habitats (Green and Short 2003), and hence the focus of this review. Eelgrass originated in the Pacific Ocean some 8–20 million years ago and colonized the Atlantic via the Arctic in repeated contemporary gene flow events. The result is minimal genetic differentiation among the three coasts (Olsen et al. 2016), which are thus considered the same phyloregion (Daru et al. 2017). Nevertheless, each bioregion exhibits unique oceanographic and hydrodynamic processes (Spalding et al. 2007; DFO 2009b), and the same species can experience a wide range of abiotic and biotic conditions and human impacts within and across regions and political jurisdictions. These variations can affect eelgrass distribution, plant morphology, habitat structure, and associated species composition as well as their response to disturbance or restoration (Cullain et al. 2018a; Namba et al. 2018; Wong 2018). These differences can further affect the biodiversity and ecosystem services eelgrass meadows provide (Hughes et al. 2004; Heck et al. 2008; Wong 2018) with implications for management and conservation at different spatial scales, including federal, provincial–territorial, and local governance levels.
Here we synthesize current knowledge of eelgrass ecosystems along Canada’s coasts to assess whether variations among bioregions suggest important differences in the ecological role and management of eelgrass across Canada. Our synthesis is structured in six parts: (i) a general overview of eelgrass distribution and trends across Canada; (ii) a comparison of Atlantic, Arctic–Subarctic, and Pacific coastal environments in which eelgrass occurs; (iii) an assessment of how regional environmental regimes affect eelgrass plant morphology, phenology, and habitat structure; (iv) a summary of known faunal species assemblages associated with eelgrass meadows in the different regions; (v) a comparison of human activities and how they affect eelgrass ecosystems across regions; and (vi) a discussion of how observed differences might affect eelgrass management and conservation from coast to coast to coast. We also review existing research efforts and identify knowledge gaps that prevent meaningful comparisons in key areas.
2. Eelgrass distribution and trends across Canada
2.1. Distribution
Eelgrass has been documented in all but three Canadian marine bioregions (Fig. 1). In the Arctic and Subarctic, eelgrass inhabits Hudson Bay and James Bay (Fig. 1; Stewart and Lockhart 2004; Government of Nunavut 2010; Mathieson et al. 2010; Wilson and Lotze 2019), and James Bay historically hosted vast subtidal eelgrass meadows, suspected to be the largest in North America (Lalumière et al. 1994). Much less is known about eelgrass distribution in the higher Arctic, but it has been observed near settlements in the Northwest Territories and Nunavut, with the most northern observation at Grise Fiord in Nunavut in the Eastern Arctic (∼76°N; Government of Nunavut 2010).
Fig. 1.

Along the Atlantic coast, eelgrass meadows are typically monospecific but can be mixed with the less abundant and primarily brackish widgeon grass (Ruppia maritima) when there is strong freshwater influence (Wong et al. 2013). In the Gulf of St. Lawrence, extensive eelgrass meadows are found along the coasts of New Brunswick, northern Nova Scotia, Prince Edward Island, Québec, and Newfoundland (Fig. 1; DFO 2009a; Schmidt et al. 2012; McIver et al. 2015; Hitchcock et al. 2017; Murphy et al. 2019). On the Scotian Shelf, eelgrass meadows are found along Nova Scotia’s Atlantic coast, including Cape Breton Island (Cullain et al. 2018a; Wong 2018), but are sparse in the Bay of Fundy, where extreme tidal heights and high sediment loads preclude eelgrass persistence in most parts (Lotze and Milewski 2004). Eelgrass meadows are also commonly found along the northeast coast of Newfoundland, but there are fewer observations on its southern coast and on the Labrador coast (Fig. 1). In contrast to other parts of Atlantic Canada, eelgrass in Newfoundland does not grow in the shallow subtidal (<1 m depth) or intertidal, where winter storms and sea ice damage eelgrass rhizomes, precluding plant growth and persistence (Rao et al. 2014), but it typically occurs at 1–6 m depth.
On the Pacific coast, intertidal and subtidal eelgrass meadows are found along the entire coastline of British Columbia but are absent from the Offshore bioregion (Fig. 1). Pacific eelgrass meadows range from small fringing meadows found on gentle slopes to large expansive meadows on tidal flats (Durance 2002). Like Atlantic eelgrass meadows, those in the Pacific are typically monospecific but can be mixed with widgeon grass under strong freshwater influence. Pacific Z. marina meadows can also be mixed with the non-native eelgrass species Z. japonica in expansive tidal flats (Shafer et al. 2014). In British Columbia, Z. japonica has been documented in the Strait of Georgia and the west coast of Vancouver Island (Mach et al. 2014). It has also been documented as far north as Alaska (Talbot et al. 2016). Some of the largest documented meadows (∼33 km2) of eelgrass (primarily Z. marina, but sometimes Z. marina and Z. japonica) are primarily intertidal and found near the Fraser River outflow in southern British Columbia (Boundary Bay; BCMCA 2011) and at the mouth of the Skeena River further north (Flora Bank; BCMCA 2011). Throughout the region, eelgrass can form vast networks of nearly continuous meadows in inlets with large shallow and gently sloped areas of suitable substrate. Notable examples of extensive networks of meadows separated by rocky substrates or unsuitable habitat with steep slopes or high wave exposure include Masset Inlet on Haida Gwaii and Clayoquot Sound on the west coast of Vancouver Island.
Mapping of specific eelgrass meadows on all three coasts of Canada has been conducted using various remote sensing techniques (e.g., satellite, benthic sonar, LiDAR) as well as aerial photography and underwater video (e.g., O’Neill and Costa 2013; Vandermeulen 2014; Barrell et al. 2015; Webster et al. 2016). These efforts tend to be site-specific, providing estimates of seagrass cover for specific meadows or regions of interest. To date, the total extent (m) and area (m2) of eelgrass on Canada’s coastlines remains unknown, although several federal agencies (Fisheries and Oceans Canada, Environmental and Climate Change Canada, Statistics Canada) are currently working towards providing these estimates.
2.2. Temporal trends
The data necessary to quantify the spatial extent and condition of eelgrass meadows and their change over time are lacking for many parts of Canada. A recent compilation of eelgrass surveys by the Canadian Environmental Sustainability Indicators Program with Environment and Climate Change Canada (ECCC, unpublished data used with permission) identified 456 eelgrass meadow locations across seven bioregions, but only 23% of these had enough observations to determine temporal trends in eelgrass biomass, extent, cover, or shoot density. Surveys with trend estimates identified 85% of eelgrass meadows as either increasing, in recovery, restored, or stable, whereas 15% showed declines. However, there was a clear disparity in the availability of surveys and trend estimates across Canada’s coasts, with only 10 trend estimates reported for the Arctic–Subarctic, 36 for the Atlantic, and 61 for the Pacific (Fig. 2). Of these, the majority (93%) of Pacific eelgrass meadows showed stable, restored, or increasing trends compared with 69% in the Atlantic, whereas a greater proportion of Atlantic eelgrass meadows showed declining (31%) trends (Fig. 2). This difference may be due to the larger number of eelgrass restoration projects along the Pacific coast (SCWG 2020), whereas only a few active restoration projects currently exist in Atlantic Canada (Mersey Tobeatic Research Institute (MTRI) and Parks Canada 2016). The majority (80%) of trend estimates for the Arctic–Subarctic identified eelgrass meadows as “in recovery” since cover of eelgrass is beginning to increase in James Bay after a major decline following hydroelectric development.
Fig. 2.

The recently compiled ECCC inventory is the first of its kind in Canada and a major step towards a national eelgrass assessment (ECCC, unpublished data used with permission). Nevertheless, there is a general lack of standardized baseline data or reference conditions; thus, any temporal trends should be evaluated with caution. Because the timelines and types of observations are not standardized across surveys, it is difficult to evaluate whether changes at individual locations and in individual measures represent stochastic variation or real long-term change from historical conditions. More and better standardized survey or monitoring efforts across Canada would help improve future trend estimates over time and across regions.
3. Spatial variation in environmental conditions affecting eelgrass
Globally, the factors controlling eelgrass growth and survival are well studied and known to be quite variable (Lee et al. 2007). Along Canada’s coasts, eelgrass inhabits shallow coastal waters that span a broad range of environmental and oceanographic conditions. As a higher plant, eelgrass requires soft sediments for its roots and rhizomes and good water quality with high light availability for photosynthesis and growth (Hemminga and Duarte 2008). Temperatures above 30 °C and salinities below 5 practical salinity units (PSU) can limit eelgrass growth and survival thereby affecting its distribution (Hemminga and Duarte 2008; Wilson and Lotze 2019). Nutrient availability is also important and can restrict eelgrass growth if too low, whereas nutrient enrichment and associated eutrophication of coastal waters can lead to light limitation and algal overgrowth (Hauxwell et al. 2003; Schmidt et al. 2012). Additionally, ice cover and scour can be important in some regions (Robertson and Mann 1984; van der Heide et al. 2009). In the following we synthesize for the first time how these key environmental drivers affect eelgrass and highlight how drivers differ within and among Canada’s coasts.
3.1. Sediments
Eelgrass persistence and growth strongly depend on the presence of suitable soft sediments, which range from muddy to sandy substrata that can be mixed with gravel, cobble, or shells (Wong 2018). Such soft sediments are found along all of Canada’s coastlines, often located in sheltered bays, inlets, and estuaries but not along exposed rocky shores (Wilson et al. 2019). Sediment chemistry is also important; eelgrass requires high oxygen content and cannot persist in anoxic sediments (Pulido and Borum 2010; Schmidt et al. 2012). The nutrient content of sediments is important for eelgrass growth (see Section 3.5.; Short 1987). High sulphide or ammonia concentrations in polluted sediments with high organic matter or in eutrophied waters can be detrimental or even toxic to eelgrass plants (van Katwijk et al. 1997; Schmidt et al. 2012; Govers et al. 2014). These properties do not vary systematically among marine bioregions or coasts, but rather from site to site depending on local oceanographic and biogeochemical conditions as well as human impacts (McIver et al. 2015; Cullain et al. 2018b; see Section 6). However, locations with longer water residence times, warmer waters, or higher population densities such as the Strait of Georgia, Gulf of Saint Lawrence, and Scotian Shelf are generally more likely to have sites with poor quality sediments.
3.2. Light availability
Irradiance (i.e., the flux of radiant energy per unit area) and particularly the photosynthetically active radiation (PAR) of the light spectrum are important for eelgrass growth and persistence (Lee et al. 2007; Hemminga and Duarte 2008). Light limitation due to natural water turbidity or depth can restrict eelgrass distribution, and increased turbidity is a major cause of seagrass decline in coastal waters worldwide (Orth et al. 2006; Waycott et al. 2009; Short et al. 2011). Across Canada’s bioregions, light availability varies with latitude, season, tides, weather patterns (e.g., cloud cover), and water colour conditions (e.g., absorption and scattering properties; Brock 1981; Kirk 2010). Differences in light regimes affect eelgrass phenology and depth limit (Olesen and Sand-Jensen 1993; Clausen et al. 2014), the allocation of photosynthate within tissues, and thus plant morphology. Shorter growing seasons at higher latitudes mean that eelgrass will depend more on morphological changes and mobilization of stored nutrients to maintain a neutral or positive carbon balance during overwintering compared with plants at lower latitudes (Burke et al. 1996; Moore et al. 1996).
At the coarsest scale, latitude affects day length, which affects light availability throughout the year (Brock 1981; Dennison and Alberte 1982). Seasonal variation in day length on the Atlantic and Pacific coasts is similar (i.e., 15.2–17.1 h at summer solstice, 6.8–8.8 h at winter solstice, for 43°–55°N) but more extreme in the Arctic (63°–73°N) where eelgrass experiences 20–24 h of daylight during the summer yet almost day-long darkness in the winter (Table 1). However, day length does not necessarily reflect the intensity of solar energy, and the cumulative time above photosynthetic light saturation, which directly affect eelgrass performance (Dennison and Alberte 1982; Olesen and Sand-Jensen 1993). At this time, local PAR measurements needed to measure these light availability parameters are not available at large scales.
Table 1.
Day length (h) | Sea surface temperature (°C) | Chlorophyll-a (mg·m−3) | Kd (m−1) | ||||||
---|---|---|---|---|---|---|---|---|---|
Coast | Bioregion | Winter | Summer | Winter | Summer | Winter | Summer | Winter | Summer |
Pacific | Strait of Georgia | 7.8–8.1 | 15.8–16.1 | 7.0 (0.3) | 17.8 (1.1) | 8.7 (5.5) | 18.6 (8.8) | 0.30 (0.07) | 0.43 (0.06) |
Southern Shelf | 7.9–8.1 | 15.8–16.1 | 8.1 (0.2) | 13.6 (0.8) | 8.0 (4.9) | 12.9 (6.3) | 0.26 (0.03) | 0.37 (0.07) | |
Northern Shelf | 7.0–7.8 | 16.1–17.0 | 7.2 (0.5) | 14.1 (0.7) | 6.1 (5.5) | 7.0 (5.1) | 0.21 (0.06) | 0.28 (0.07) | |
Arctic | Western Arctic | 2.1 | 24.0 | −1.8 (0.1) | 3.0 (3.3) | NA | 1.5 (1.9) | NA | 0.20 (0.15) |
Arctic Archipelago | 0 | 24.0 | −1.8 (0.1) | −1.2 (0.6) | NA | 1.4 (1.5) | NA | 0.17 (0.03) | |
Eastern Arctic | 0–3.9 | 20–24 | −1.8 (0.02) | 1.5 (1.5) | NA | 1.0 (0.8) | NA | 0.15 (0.03) | |
Hudson Bay Complex | 3.6–7.6 | 16.3–20.4 | −1.8 (0.03) | 4.6 (3.2) | 0.7 (0) | 2.8 (3.8) | NA | 0.25 (0.17) | |
Atlantic | Newfoundland–Labrador Shelves | 6.8–8.3 | 15.6–17.1 | −1.1 (0.8) | 11.4 (4.1) | 0.7 (0.5) | 2.4 (3.5) | 0.13 (0.01) | 0.19 (0.06) |
Scotian Shelf | 8.4–8.8 | 15.2–15.5 | 1.7 (1.6) | 16.7 (1.8) | 3.5 (1.7) | 4.2 (4.4) | 0.34 (0.21) | 0.30 (0.13) | |
Gulf of Saint Lawrence | 7.6–8.5 | 15.4–16.3 | −1.1 (0.4) | 16.5 (2.8) | 2.6 (2.8) | 11.0 (10) | 0.19 (0.07) | 0.35 (0.14) |
Note
Day length is the number of daylight hours. Sea surface temperature (SST) averaged within 10 km of the coast (NOAA ERD and CoastWatch West Coast Regional Node 2019); note that SST is not taken directly within eelgrass meadows. Chlorophyll-a concentration averaged within 10 km of the coast (NASA/GSFC OBPG 2019). Attenuation coefficient of downwelling irradiance (Kd) integrated over photosynthetically active radiation (PAR) averaged within 10 km of the coast (GlobColour Project 2019). Data presented as means (± standard deviation, SD) for winter (coldest month = February) and summer (warmest month = August) from 2010 to 2019 (SST and Chlorophyll-a) and from 1997 to 2019 (Kd).
Regional differences in tidal regimes also affect light availability. On the Pacific coast, mixed semidiurnal tides create strong seasonality in light availability. Between September and March equinoxes, high tides occur during the day during limited daylight hours and low tides are at night. Consequently, Pacific eelgrass in the mid to low intertidal receive virtually no exposure to moderate or high light levels during fall and winter. The pattern is reversed from March to September when low tides occur in the daytime. Conversely in the Arctic, low tides occur during the day during the dark half of the year and high tides occur during the summer midday. On the Atlantic coast, semidiurnal tidal regimes mean that eelgrass experience both high and low tides during daylight hours in the winter and summer months, although irregularities occur in some regions (McIver et al. 2015).
One gauge of light availability is the diffuse attenuation coefficient of downwelling irradiance (Kd), a measure of how quickly light is absorbed as it travels through the water column (Kirk 2010). High Kd levels mean that light cannot penetrate as deeply; thus, Kd can be an indicator of the depth limit of eelgrass (Duarte 1991; Gattuso et al. 2006; Ralph et al. 2007). Both natural and human factors can increase Kd (Gallegos and Moore 2000; Gallegos 2001), and generally Kd is higher in nearshore waters where land-runoff and human influences are stronger (Fig. 3). In the Supplementary Material 1 we show in detail how we assessed and processed Kd for the studied areas. Almost all bioregions show a seasonal signal with higher Kd from January to April (Fig. S1), likely reflecting either continental spring runoff, with higher concentrations of coloured dissolved organic matter and suspended inorganic particles, or phytoplankton spring blooms with higher concentration of pigments. The Strait of Georgia and the Gulf of Saint Lawrence bioregions show the highest seasonal variability in Kd and, together with the Scotian Shelf, present consistently higher Kd values throughout the year. In contrast, Kd in the outer coast Pacific and Newfoundland–Labrador bioregions show smaller seasonal differences, possibly indicating a predominant open ocean influence. The Arctic bioregions show lower mean Kd values, with peak values in June and July possibly reflecting sea ice melting processes. These results suggest that the depth limits of eelgrass meadows may be shallower in the Strait of Georgia, Gulf of Saint Lawrence, and Scotian Shelf bioregions given the persistently higher Kd values in these bioregions.
Fig. 3.

3.3. Temperature
Eelgrass has a wide temperature tolerance from −1.5 to ∼30 °C and grows generally well between 10 and 25 °C (optimum temperature for photosynthesis ∼23 °C, Lee et al. 2007). Reduced growth and increased mortality occur above ∼25 °C (Nejrup and Pedersen 2008). High temperatures also increase the light requirements of eelgrass by increasing respiration and decreasing photosynthesis rates (Beca-Carretero et al. 2018). Consequently, high temperatures can exacerbate the effects of light limitation and reduce plant resilience to sustained stress by negatively affecting carbon storage (Marsh et al. 1986; Staehr and Borum 2011). Production of flowering shoots has been observed across the temperature tolerance range of eelgrass with peak seed maturation at ∼20 °C (Blok et al. 2018). However, sexual reproduction in eelgrass is poorly studied in Canada (Blok et al. 2018). Eelgrass can successfully overwinter under sea ice (Lalumière et al. 1994; Wong et al. 2013; Olesen et al. 2015) and has some resistance to freezing (Biebl and McRoy 1971).
Water temperature regimes differ substantially across Canada’s bioregions (Fig. 3, Table 1). They depend on latitude and seasonal light regimes and the influence of warm- and cold-water influx from currents (e.g., Gulf Stream and Labrador Current on the Atlantic coast), extensive upwelling (e.g., on the Pacific and Atlantic coasts), tidal mixing, and ice melting. Given its wide temperature tolerance, conditions are generally suitable for eelgrass to grow and persist across all bioregions, although optimal growing conditions are rarely reached in the Arctic because of low summer temperatures (Table 1). Along all three coasts, water temperatures can rise rapidly in shallow bays during summer days, particularly when light intensity is high, water depth is shallow, and flushing is low, thus exceeding 25 °C on the Pacific and Atlantic coasts (Wong et al. 2013; Krumhansl et al. 2020) and 20 °C in James Bay (Lalumière et al. 1994). Intertidal eelgrass also often experiences higher temperatures and face additional stress from desiccation during low tide while exposed to air (Boese et al. 2003; Yang et al. 2013). During winter, water temperatures along the Pacific coast typically stay above freezing, with some ice in shallow bays, whereas eelgrass regularly persists in water as low as −1.5 °C and under sea ice in the Arctic and parts of the Atlantic bioregions (Table 1).
3.4. Salinity
Eelgrass can tolerate a broad range of salinities, from 5 PSU in brackish estuaries to 35 PSU in fully marine waters across Canada’s bioregions (Lee et al. 2007). Optimal salinities for growth and reproduction are typically >15 PSU, but adult plants can tolerate periods of low salinity (<5 PSU) although seedling germination and shoot survival are diminished (Nejrup and Pedersen 2008). Within regions, the freshwater effluent of major river basins, such as the Fraser and Skeena rivers in British Columbia, the La Grande River in James Bay, and the St. Lawrence River in the Gulf of St. Lawrence, creates broad salinity gradients over large spatial scales (Granskog et al. 2011) and restricts eelgrass distribution in low-salinity areas (Lalumière et al. 1994; Nejrup and Pedersen 2008).
3.5. Nutrient availability
Sufficient nutrients are essential to eelgrass growth, particularly the availability of nitrogen which is often limiting in marine waters. Nutrient availability can affect several aspects of plant and bed structure, including leaf length and canopy height, shoot density, above and belowground biomass, as well as tissue nutrient content and epiphytic growth (Touchette et al. 2003; Schmidt et al. 2012). In coastal waters, nutrient availability is regulated in large part by the physical processes of ocean circulation, such as upwelling and mixed-layer dynamics and atmospheric deposition (Hessing-Lewis and Hacker 2013), but can be altered by land- and marine-based human activities that enhance nutrient loading at local to regional scales (McIver et al. 2015, see Section 6).
General nutrient availability differs among Canada’s oceans, with the Pacific coast being characterised by strong upwelling in the spring, which brings cold, nutrient-rich waters to the surface in the summer (Foreman et al. 2011). Such upwelling events are less common on the Atlantic coast, though some wind-driven upwelling can occur periodically during spring and summer (Garrett and Loucks 1976; Ings et al. 2008; Krumhansl et al. 2020). As a result, surface-water chlorophyll-a concentrations are generally more elevated on the Pacific coast and particularly in the Strait of Georgia than elsewhere in Canada (Bristow et al. 2017). Thus, nutrient availability and limitation differs among Canada’s coasts and bioregions, which will influence the local or regional effects of nutrient loading and eutrophication (see Section 6).
3.6. Ice cover
Ice cover represents a significant physical disturbance that may shape the distribution and phenology of eelgrass on Canada’s Atlantic and Arctic coasts but rarely occurs on the Pacific coast. Generally, eelgrass can survive under ice despite reduced light availability (McRoy 1969; Wong et al. 2013). However, ice can be damaging for intertidal and shallow subtidal eelgrass meadows (<1 m high tide), where ice scour can remove eelgrass leaves and the majority of aboveground biomass (Robertson and Mann 1984). Eelgrass plants in these conditions often allocate a greater proportion of production to their underground rhizome structure, allowing them to remain well anchored in the sediment and survive the winter (Robertson and Mann 1984). This is not the case for new seedlings which are less resistant to ice scour (Robertson and Mann 1984). On the northeast coast of Newfoundland, damage to eelgrass meadows can also result from the shoreward drifting of pack ice during spring, which occurs most years (R.S. Gregory, personal observation, 2020). With climate warming, the threat of ice scour and shading may decrease along many parts of the temperate Atlantic coast over the next century but will continue in the Arctic (Bush and Lemmen 2019; Wilson and Lotze 2019).
4. Spatial variation in eelgrass life history, phenology, and plant and bed structure
Eelgrass meadows can exhibit both perennial or annual life-history strategies or a mix of the two (Keddy and Patriquin 1978; Jarvis et al. 2012). Perennial meadows persist over winter and generally dominate along the Atlantic and Pacific coasts and in James Bay (Phillips et al. 1983a; Schneider et al. 2008; Warren et al. 2010; Wong et al. 2013), though recently many annual meadows have also been observed in James Bay. In the summer, perennial meadows display a mix of both sexual and asexual reproduction (via new lateral shoot growth; Olesen 1999), although sexual reproduction usually occurs in <10% of shoots (B. Vercaemer, unpublished data; E.M. Adamczyk, unpublished data). In contrast, annual meadows depend on sexual reproduction, where reproductive shoots produce seeds that overwinter and germinate into new shoots in the next growing season. Annual plants die after seed maturation and there is no belowground or aboveground biomass present over the winter in a meadow with an annual life history. This strategy can be advantageous in areas of high environmental disturbance (e.g., high water temperature, ice cover, or sediment resuspension; Kim et al. 2014). A few annual eelgrass meadows have also been identified in Nova Scotia in intertidal and shallow subtidal areas that are influenced by ice cover in the winter (Robertson and Mann 1984).
Along all three coasts, eelgrass follows a similar seasonal pattern of growth and reproduction (phenology). High growth rates occur from spring until mid-summer, when shoot density and biomass reach a maximum (Robertson and Mann 1984; Clausen et al. 2014). Sexual reproduction begins in early to mid-spring with flowering shoots present until late summer (B. Vercaemer, unpublished data; Blok et al. 2018). Partial senescence of vegetative shoots begins in late summer, reducing shoot density and biomass and, along with fall and winter storm disturbance, producing large wrack deposits on nearby shorelines. Low shoot density and aboveground biomass persist over winter, when low light conditions restrict photosynthesis. Carbohydrates stored in the rhizomes during the growing season are used over the winter to maintain carbon balance (Wong et al. 2020).
Despite distinct oceanographic and climate regimes in the Pacific and Atlantic bioregions, eelgrass phenology in these regions is typical for temperate seagrasses and largely driven by temperature and light (Phillips et al. 1983b; Blok et al. 2018). In contrast, Arctic and Subarctic eelgrass phenology is influenced by coastal sea-ice, which delays the start of the growing season until after ice break-up in the summer. Phenology also depends on local environmental conditions. For example, in Nova Scotia, very shallow meadows in protected areas and muddy sediments tend to experience larger seasonal changes in shoot density and biomass than deeper meadows in more exposed areas with sandy sediments (M.C. Wong, unpublished data).
In addition to seasonal changes, environmental heterogeneity at all scales influences eelgrass survival and production, thereby affecting shoot density, leaf length, and canopy height, and shoot (aboveground) and root and rhizome (belowground) biomass. In each bioregion, high variability occurs in these structural properties of eelgrass plants and meadows (Table 2), which depend on water depth, sediment properties, light conditions, and other environmental variables (see Section 3; Olesen and Sand-Jensen 1994; Schmidt et al. 2012; Ruesink et al. 2018). For example, eelgrass can respond to: reduced light conditions by increasing leaf length to capture more light or decreasing leaf length to reduce respiratory demand, high water currents by increasing belowground biomass to strongly anchor plants in the sediments, and silty and muddy sediments by reducing belowground biomass and growing rhizomes at the sediment surface for more oxygen and less sulphide intrusion (Penhale and Wetzel 1983; Short et al. 1995; Pedersen et al. 2004; Wong et al. 2020). Recent studies on both the Atlantic and Pacific coasts have shown significant relationships between eelgrass plant and bed metrics and environmental conditions (Thom et al. 2003; Cullain et al. 2018a; Namba et al. 2018; Wong 2018) as well as anthropogenic impacts, such as nutrient enrichment (Schmidt et al. 2012; McIver et al. 2019) and sediment loading (Stark et al. 2020). Data for the Arctic bioregions is limited to the subarctic region of James Bay in the Hudson Bay Complex (Table 2).
Table 2.
Bioregion | Sampling depth (m) | Shoot density (number·m−2, ±SD) | Canopy height (cm, ±SD) | Aboveground biomass (g dry weight·m−2, ±SD) | Belowground biomass (g dry weight·m−2, ±SD) | Reference |
---|---|---|---|---|---|---|
Pacific Coast | ||||||
Strait of Georgia | 0–1.0 | 176 (64) | 61 (7.6) | 126.5 (28.8) | — | Knight et al. 2015 |
0.5–3.0 | 265 (80) | — | 305 (59) | 71 (38) | Röhr et al. 2018 | |
0.5–1.0 | 679 (564) | 63 (31) | 124 (69) | — | E.M. Adamczyk, unpublished data | |
0.5–5.0 | 74 (38) | 46 (31) | 127 (96) | — | Stark et al. 2020 | |
Southern Shelf | 0–0.5 | 158 (62) | 67 (18.7) | 47 (26) | 16 (12) | Postlethwaite et al. 2018 |
∼1.0–3.0 | 111 (24) | — | — | — | Whippo et al. 2018 | |
0.5–5.0 | 260 (227) | 56 (25) | 78 (33) | — | Stark et al. 2020 | |
0.5–8.0 | 173 (90) | 90 (21) | 122 (68) | — | E.M. Adamczyk, unpublished data | |
0.5–3.0 | 211 (43) | — | 139 (42) | — | Röhr et al. 2018 | |
Northern Shelf | 0.5–5.0 | 311 (200) | 48 (25) | 157 (67) | — | Stark et al. 2020 |
0.5–3.0 | — | — | — | 27 (19) | Röhr et al. 2018 | |
NA | — | 58 (5) | — | — | Hessing-Lewis et al. 2018 | |
2.0–7.0 | 194 (171) | 88 (35) | 134 (86) | — | M.I. O’Connor, unpublished data | |
1.0–8.0 | — | 129 (4.8) | — | — | Olson 2017 | |
Atlantic coast | ||||||
Newfoundland–Labrador Shelves | 2.2–2.5 | 121 (60) | 39 (13) | 101 (50) | 144 (77) | Cullain et al. 2018a |
Scotian Shelf | 1.8–4.9 | 233 (140) | 60 (21) | 223 (146) | 427 (210) | Schmidt et al. 2011 |
1.4–4.9 | 161 (111) | 50 (22) | 225 (163) | 329 (270) | Cullain et al. 2018a | |
1.0–8.0 | 623 (400) | 46 (14) | 286 (175) | 470 (472) | Wong 2018 | |
Gulf of Saint Lawrence | 0.75–1.0 | 380 (257) | 48 (21) | 171 (109) | 426 (295) | Schmidt et al. 2012 |
0.75–1.5 | 112 (62) | 37 (14) | 113 (47) | 208 (101) | Cullain et al. 2018a | |
0.3–0.6 | 364 (247) | — | 76 (69) | — | Skinner et al. 2013 | |
Hudson Bay Complex | 1.0 | 694 (185)a | — | 218 (69)a | — | Lalumière et al. 1994 |
1.0 | 206 (37)a | — | 53 (21)a | — | Lalumière et al. 1994 | |
1.0 | 230 (75) | 52 (11) | — | — | M.I. O’Connor et al., unpublished data | |
1.0 | 300 (80) | 50 (4) | — | — | M.I. O’Connor et al., unpublished data |
Note
All measurements were taken during summer months (May–September) and are represented as mean (± standard deviation, SD). Note that surveys employed different methods and metrics may not be directly comparable.
a
Value in parentheses is the 95% confidence interval.
5. Species assemblages supported by eelgrass habitats
Eelgrass meadows provide food, shelter, settlement substrate, breeding, spawning, and nursery habitat for a wide range of flora and fauna (Laurel et al. 2003a; Robinson et al. 2011; Schmidt et al. 2017; Wong 2018). Different zones in eelgrass meadows support different species assemblages, such as epiphytic assemblages on eelgrass leaves, pelagic assemblages within and above the eelgrass canopy, benthic assemblages on or near the sediment, and infaunal assemblages within the root and rhizome system (Fig. 4). Epiphytic micro- and macroalgae (e.g., diatoms, seaweeds), invertebrates (e.g., hydroids, bryozoans), and microbial assemblages growing on eelgrass blades provide food for many kinds of smaller mobile invertebrates such as amphipods, copepods, isopods, shrimps, gastropods, and others (Schmidt et al. 2011; Whippo et al. 2018; Segovia et al. in review). Many small and juvenile fish and larger invertebrates (e.g., lobster, crabs) feed on this variety of algae, smaller invertebrates, and detritus in eelgrass meadows. Fishes include year-round residents such as sculpins and gunnels (Robinson et al. 2011; Huang et al. 2015; Iacarella et al. 2018) and others such as shiner perch, stickleback, rockfish, cod, salmon, eel, and herring that use eelgrass during parts of their life cycle (Bradbury et al. 2008; Copeman et al. 2009; Amundrud et al. 2015; Chalifour et al. 2019; Olson et al. 2019). Larger vertebrates such as sea lions and otters as well as herons, geese, ducks, and other birds are often observed in eelgrass meadows and provide trophic linkages between eelgrass meadows and other habitats when they forage in multiple eelgrass meadows (Seymour et al. 2002; Huang et al. 2015; Hessing-Lewis et al. 2018). Eelgrass primary and secondary production thus supports food webs that span local (within meadow) to regional scales and cross four or more trophic levels.
Fig. 4.

An important role of eelgrass habitats is to act as a refuge from predation, particularly for small and vulnerable fishes and invertebrates (Linehan et al. 2001; Laurel et al. 2003b; Gorman et al. 2009; Olson et al. 2019). For example, eelgrass meadows support higher densities of juvenile cod (Warren et al. 2010) and juvenile Pacific salmon (Sharpe et al. 2019) and higher growth rates of juvenile fish species than unvegetated patches (Tupper and Boutilier 1995; Renkawitz et al. 2011), which can result in higher survivorship (Ryan et al. 2012) and recruitment to fisheries (Gregory et al. 2019; Cooke et al. unpublished data). Eelgrass meadows also enhance overall lifetime production of fishes relative to adjacent bare sediment (Wong and Dowd 2016). Generally, there is higher species richness and abundance within eelgrass meadows compared to adjacent unvegetated habitat on the Atlantic and Pacific coasts (Joseph et al. 2006; Burd et al. 2008; Schmidt et al. 2011; Cote et al. 2013; Wong 2018; Whalen et al. unpublished data). Although understudied, the same pattern likely exists in the Arctic as it is commonly observed in seagrass ecosystems worldwide (Guidetti 2000). Apart from eelgrass presence, the structure of eelgrass meadows (shoot density, canopy height, biomass, and patch fragmentation), environmental conditions, landscape context, and species interactions within and around meadows can influence the composition and abundance of associated assemblages (Thistle et al. 2010; Cullain et al. 2018a; Iacarella et al. 2018; Whippo et al. 2018; Wong and Kay 2019; Stark et al. 2020).
5.1. Comparison of assemblage surveys across bioregions
In all regions, eelgrass food webs have a similar cast of characters in terms of the higher order taxonomic and functional groups represented (Fig. 4). Yet beyond this broad characterization, more specific food-web comparisons are difficult to make. Although several field surveys on the Atlantic and Pacific coasts and ongoing work in James Bay, have assessed eelgrass-associated species assemblages, they often focused on different parts of the assemblages (e.g., infauna or benthic fauna), different species groups (e.g., invertebrates or fish), different size groups (macro- or meiofauna) or used different sampling techniques. These differences highly influence the results and make surveys difficult to compare within or across bioregions. Disparities among surveys are often driven by differing research goals, site characteristics, and a lack of standardised sampling protocols (see Appendix A for details). Organized biodiversity surveys coordinated across regions would resolve this issue. In the following we therefore only highlight some general patterns that can be derived from existing studies on the Atlantic and Pacific coasts, whereas assemblage studies are largely lacking from the Arctic.
Environmental factors appear to be important in explaining some spatial variation in eelgrass-associated assemblages across Atlantic bioregions (Cullain et al. 2018a; Wong 2018; Wong and Kay 2019), but this is less evident for the Pacific (Whippo et al. 2018; Stark et al. 2020). In Atlantic Canada, several surveys using consistent sampling methods revealed distinct differences within and across bioregions that could be linked to environmental conditions, particularly water temperature, and eelgrass bed structure (e.g., shoot density and canopy height) (Schmidt et al. 2017; Cullain et al. 2018a; Namba et al. 2018). These studies from low-impacted eelgrass meadows suggest natural regional differences in habitat provision and ecosystem structure that could inform spatial management and conservation.
On the Pacific coast, meadows along the entire coast of British Columbia share the same regional fish and invertebrate fauna, with no clear latitudinal signal in eelgrass-associated species assemblages (Robinson et al. 2011; Siegle et al. 2014; Iacarella et al. 2018; Stark et al. 2020). Several studies found that epifaunal invertebrate biodiversity varied substantially within and among eelgrass meadows (Whippo et al. 2018; Stark et al. 2020), yet this variation could not be clearly explained by bioregion or abiotic variables, such as temperature, nutrients, or salinity. Instead, species interactions, priority effects (i.e., processes associated with spatial community assembly), and landscape context are likely driving some of the observed spatial patterns (Whippo et al. 2018; Stark et al. 2020). Most studies in the Pacific and Atlantic bioregions have focused on evaluating taxonomic diversity of eelgrass-associated assemblages, which is not necessarily correlated with functional diversity (Wong and Dowd 2015). Functional diversity is more closely linked to ecosystem functioning (Hooper et al. 2005; Norling et al. 2007), which has strong implications for management and conservation of eelgrass ecosystems (Stuart-Smith et al. 2013). On the Atlantic coast, functional diversity patterns of benthic invertebrates and fish assemblages were found to change with environmental conditions and characteristics of eelgrass meadows (Cote et al. 2013; Wong and Dowd 2015; Wong and Kay 2019). In the Pacific, species turnover has also been observed among eelgrass meadows, although similar species often replace each other and the different assemblages do not clearly correlate with environmental variation. Further studies on functional diversity and by extension ecosystem functioning in Canada’s eelgrass ecosystems could provide important insight for their management and conservation (Díaz and Cabido 2001; Micheli and Halpern 2005; Petchey and Gaston 2006).
The food web structure of eelgrass ecosystems has also been poorly studied across Canada’s bioregions. Comprehensive food webs for eelgrass ecosystems have only been constructed for the Scotian Shelf and Gulf of St. Lawrence (Coll et al. 2011; Schein et al. 2013). Coll et al. (2011) found generally similar food web structure between Scotian Shelf and Gulf of St. Lawrence eelgrass meadows, but only for meadows experiencing low human impacts. Furthermore, the properties used to characterize food web structure (i.e., number of trophic groups, connectance, etc.) were comparable among eelgrass meadows in Atlantic Canada and tropical seagrass meadows, suggesting that despite differences in environmental conditions and species assemblages, general food web structure may not differ strongly across bioregions. However, further studies, ideally as part of a coordinated biodiversity assessment program, are needed to compare eelgrass associated food webs across Atlantic, Arctic, and Pacific bioregions.
6. Human impacts on eelgrass ecosystems
As nearshore habitats, eelgrass ecosystems are vulnerable to human activities occurring both on land and in coastal waters (Orth et al. 2006; Waycott et al. 2009), such as coastal development, overwater structures, nutrient loading, aquaculture, commercial fishing activities, and invasion by non-native species, among others (Murphy et al. 2019). Together, these activities can increase pollution and sediment runoff, alter water circulation and freshwater inputs, cause shading, and physically damage eelgrass (Eriander et al. 2017; Lefcheck et al. 2018; Kelly et al. 2019). Additionally, eelgrass is affected by climate change, including rising water temperature, altered salinity regimes, sea level rise, increased carbonate concentration associated with ocean acidification, and increased turbidity from storms (Short and Neckles 1999; Wilson and Lotze 2019).
Although each of Canada’s marine bioregions is influenced by human activities, there is considerable variation in the types and strengths of impacts most prevalent among regions (Iacarella et al. 2018; Murphy et al. 2019). Furthermore, differences in physiological and evolutionary adaptation to environmental differences outlined in Section 3 may cause differences in eelgrass vulnerability to human activities across regions. So far, research on the response of Canadian eelgrass and associated fauna to cumulative human activities is limited, although some studies have examined the effects of eutrophication (Coll et al. 2011; Schmidt et al. 2012, 2017; Schein et al. 2013; Hitchcock et al. 2017; McIver et al. 2019), non-native species (Garbary et al. 2014; Matheson et al. 2016; Mach et al. 2017), aquaculture (Skinner et al. 2013; Cullain et al. 2018b), coastal development (Laurel et al. 2003a; Iacarella et al. 2018), and climate change (Wilson and Lotze 2019). In this section, we assess regional variation in better-documented human activities and compare how eelgrass ecosystems across Canada’s coasts and marine bioregions might respond to these impacts.
6.1. Coastal development
Eelgrass in the Atlantic and Pacific bioregions are influenced by a wide range of coastal development impacts, while those in the Arctic are currently not subject to the same magnitude of coastal modifications. Development of shoreline and overwater structures (e.g., marinas, wharves, breakwaters, causeways, and aquaculture facilities) and alteration of natural land to urban or agriculture land use influence river flow, sediment movement, and nutrient–pollution input in coastal ecosystems (Lee et al. 2006; van Katwijk et al. 2011; Rehr et al. 2014; Quiros et al. 2017). These changes can directly affect eelgrass meadows by burying them, changing water depth, altering salinity, or reducing water clarity and quality (Erftemeijer and Lewis 2006; Eriander et al. 2017). Coastal development can also affect the faunal communities inhabiting eelgrass meadows. For example, biotic homogenization (i.e., loss of beta-diversity) of faunal assemblages has been observed in Pacific eelgrass meadows with increased coastal construction and presence of overwater structures (Iacarella et al. 2018).
Some types of coastal development impacts differ between bioregions. For example, impacts from the logging industry are of particular concern in Pacific bioregions, both from conversion of natural land and impacts from marine log transportation and storage (Sedell et al. 1991). Even after log storage areas have been decommissioned, recovery of eelgrass can be slow or impossible as wood debris leads to accumulation of hydrogen sulphide in sediments (Pease 1974; Sedell et al. 1991). On the Atlantic coast, the logging industry rarely uses ocean storage and transport which is therefore of less concern for eelgrass. However, conversion of natural land to forestry operations is prevalent throughout Atlantic Canada with concern for terrestrial-derived sediment input to Atlantic eelgrass meadows.
While Arctic and Subarctic eelgrass meadows are currently not subject to the same magnitude of coastal modifications as Pacific and Atlantic eelgrass meadows, they are being increasingly influenced by shoreline and hydroelectric development. In James Bay, an eelgrass meadow once described as the most extensive in North America (Lalumière et al. 1994) is now thought to be a fraction of its historical extent. While the exact cause of decline remains unresolved, one presumed cause is the change in hydrology related to the diversion of rivers to generate hydropower. Increasing demands for power and clean energy are expected to increase hydroelectric development, causing downstream shifts in freshwater inputs to coastal systems and impacting eelgrass ecosystems.
6.2. Nutrient loading
Generally, areas with extensively modified land cover, dense coastal inhabitation, and industrial development show higher human-derived nutrient loading to coastal waters than areas without these stressors (Bricker et al. 2008). In Canada, such areas are more commonly found in parts of the Atlantic and Pacific than Arctic coastlines. For example, in coastal waters around Prince Edward Island, nitrogen loading is dominated by agricultural run-off from fertilizer use (Bugden et al. 2014), but in New Brunswick and Nova Scotia it is by atmospheric deposition and septic systems, and in some bays it is by aquaculture activities (McIver et al. 2015, 2018; Nagel et al. 2018). On average, human-derived nitrogen loading is higher in the Gulf of St. Lawrence than on the Scotian Shelf (Murphy et al. 2019). Meanwhile, in the Pacific, anthropogenic nutrient loading is expected to be highest in the Strait of Georgia and low in the Northern and Southern Shelf bioregions, given the Strait of Georgia’s extensive coastal development, high human population densities in Vancouver and Victoria, agricultural run-off from the Fraser Valley, and untreated sewage outfalls (Mackas and Harrison 1997; Roberts et al. 2014).
In addition to the amount of anthropogenic nitrogen loads, the response of eelgrass meadows to excess nitrogen input also depends on local and regional characteristics, such as the natural supply of nitrogen and exchange rate with the open ocean (Sutton et al. 2013). For example, eelgrass meadows on the Pacific coast have likely experienced fewer eutrophication symptoms (macroalgal blooms, overgrowth of epiphytic algae on blades, etc.) compared with Atlantic meadows since Pacific bays and estuaries are highly influenced by ocean upwelling events that are the dominant source of dissolved nitrogen (Sutton et al. 2013). Though few experimental tests have explored the effects of nutrient addition on eelgrass in Pacific bioregions, and all these have been done within the Fraser River plume, there remains no strong evidence for negative effects of anthropogenic nutrient loading on eelgrass growth or size (Ruesink et al. 2018). However, increased epiphyte load on eelgrass has been observed in meadows subjected to increased local-scale human activities in the Pacific bioregions (Iacarella et al. 2018). Future human population growth and climate change could alter the nutrient regime, and the effects of anthropogenic nutrients, on the west coast. In contrast, Atlantic eelgrass meadows are likely more susceptible to the effects of anthropogenic nitrogen loading. Generally, Atlantic bays and estuaries are less influenced by ocean upwelling and have lower background levels of nitrogen. With increased anthropogenic nutrient loading, studies have observed decreased eelgrass cover and biomass, reduced fish and invertebrate species richness, and evidence for overall food web degradation (Coll et al. 2011; Schmidt et al. 2012, 2017; Coffin et al. 2018; van den Heuvel et al. 2019). It has been estimated that upwards of 64% of eelgrass meadows in Nova Scotia, New Brunswick, and Prince Edward Island are at risk of decline due to excess human-derived nitrogen loading (Murphy et al. 2019). In contrast, many eelgrass meadows in Newfoundland are currently increasing or stable (Fig. 2) and have experienced lower overall nutrient loads due to declining rural population levels in the past three decades (Statistics Canada census figures 1991–2016), whereas others are exposed to the increasing aquaculture industry.
6.3. Non-native species
Several non-native invertebrate species inhabit eelgrass meadows along the Pacific and Atlantic coasts, with similar species on both coasts (Williams 2007). These include invasive biofouling species such as the solitary clubbed tunicate (Styela clava), colonial golden star (Botryllus schlosseri), and violet tunicates (Botrylloides violaceus) (Williams 2007). Fouling by tunicates can decrease eelgrass growth and survival by reducing light reaching the leaves and by breaking leaves and shoots (Wong and Vercaemer 2012). In Atlantic Canada, coastal bays in the Scotian Shelf region have significantly higher extent of invasive biofouling tunicates compared with the Gulf of St. Lawrence or Newfoundland (Carman et al. 2019; Murphy et al. 2019). A formal analysis has not been conducted to compare biofouling invasion extent between Pacific and Atlantic bioregions, and limited work conducted in James Bay has not revealed biofouling invasions sub-Arctic eelgrass meadows. However, a predicted northward range expansion of most invasive tunicates on both the Atlantic and Pacific coasts is expected to increase the invasion extent in northern regions in the future (Lowen and DiBacco 2017; Lins et al. 2018). Furthermore, increased shipping and development of harbours and terminals in the Arctic combined with warming conditions are expected to increase the rate of introduction of non-native species in Arctic waters.
The European green crab (Carcinus maenas) is another non-native invertebrate found throughout eelgrass habitats on the Atlantic and Pacific coasts but not in the Arctic (Klassen and Locke 2007). Green crabs first arrived in British Columbia several decades later (in 1999) than on the Scotian Shelf (1951) and in the Gulf of St. Lawrence (1941), which have experienced multiple invasion events following the initial introduction (Roman 2006; Klassen and Locke 2007). Populations are currently well established in the Southern and Northern Pacific Shelf bioregions (Gillespie et al. 2015) and have only recently been recorded in the Strait of Georgia (2019) and in Haida Gwaii (2020) (B.R. Howard, DFO, personal communication, 2020). Documented evidence of their arrival in Placentia Bay, Newfoundland, is also relatively recent (2007 in North Harbour; Matheson et al. 2016). By disrupting sediment as they bury themselves and by damaging eelgrass roots and rhizomes as they forage, green crabs can negatively impact eelgrass survival and growth. High densities of green crabs have been experimentally shown to cause sharp and rapid declines in some eelgrass meadows on the Atlantic (Malyshev and Quijón 2011; Garbary et al. 2014; Matheson et al. 2016) and Pacific coasts (Howard et al. 2019). In contrast, evidence for significant negative effects of green crab invasion on eelgrass-associated assemblages is mixed. Sharp declines in eelgrass-associated fish communities have been linked to reduction in eelgrass cover caused by green crabs in Newfoundland (Morris et al. 2011; Matheson et al. 2016), whereas negative impacts of green crabs on biomass or community composition of benthic invertebrates or fishes are not apparent in eelgrass meadows in British Columbia or on the Scotian Shelf (Wong and Dowd 2016; Schmidt et al. 2017; Howard et al. 2019).
The dwarf eelgrass Zostera japonica itself is a non-native eelgrass species that was introduced from the northwestern Pacific to the Canadian Pacific coast in 1974 (Harrison and Bigley 1982). It is currently absent along the Arctic and Atlantic coasts. In the Pacific bioregions, it hosts a similar suite of eelgrass-associated species as Z. marina (Knight et al. 2015). Z. japonica is distributed higher in the intertidal zone than Z. marina and is therefore not regarded as a nuisance species as it expands available eelgrass habitat (Mach et al. 2014; Knight et al. 2015).
6.4. Climate change
Climate change is expected to change a number of physio-chemical characteristics of coastal waters with expected impacts on eelgrass ecosystems (Bush and Lemmen 2019). Changes in light availability driven by other human impacts (e.g., nutrient loading, overwater structures, etc.) may be exacerbated by climate-driven increases in storm frequency and severity that can cause sediment run-off and increase water turbidity and eelgrass smothering (Carlson et al. 2010; Curry et al. 2019). Changes to hydrology, freshwater input, and the timing of snow and ice melt will be particularly strong in the Arctic and sub-Arctic (Bonsal et al. 2019), although there are very few baseline data for eelgrass meadows in James Bay and elsewhere in the Canadian Arctic.
Increasing uptake of atmospheric carbon dioxide (CO2) is resulting in decreased pH (ocean acidification) in all of Canada’s marine bioregions (Bonsal et al. 2019). Although increased CO2 may actually enhance eelgrass photosynthetic rate and productivity (Koch et al. 2013; Zimmerman et al. 2017; Egea et al. 2018), eelgrass ecosystems are still vulnerable given that many of the inhabitants, such as bivalves and crabs, are sensitive to ocean acidification (Waldbusser and Salisbury 2014; Bednaršek et al. 2020).
A decrease in salinity in North Atlantic waters (Greene and Pershing 2007), the Gulf of St. Lawrence, and along the Pacific (Cummins and Masson 2014) is predicted with climate change as sea ice melts, patterns of precipitation change, and the timing of spring meltwater shifts (Long et al. 2016; Curry et al. 2019). Decreased salinity may negatively affect Canadian eelgrass meadows in some bays or estuaries, as salinities lower than 20 PSU have been associated with slower growth, higher mortality, and lower seedling establishment (Pan et al. 2011; Salo and Pedersen 2014; Xu et al. 2016). Still, observations suggest that eelgrass can grow in salinities as low as 5 PSU (Duffy et al. 2015) and there is evidence that eelgrass populations can adapt to lower salinity conditions, although this can cause changes in morphology, density, and at very low salinities, reductions in reproduction and subsequent mortality (Salo et al. 2014).
Long-term ocean warming is not expected to be a serious threat for eelgrass in Canada because temperatures in most of Canadian marine waters are below the optimum for eelgrass growth. Instead, future warming over the 21st century is projected to result in northern range expansions of eelgrass across Canada and into the Arctic (Wilson and Lotze 2019). Air and ocean warming may also increase eelgrass growth by lengthening the growing season through effects on the timing of ice melt and refreeze. Nevertheless, there are major concerns with the effects of short-term extreme temperature events or heat waves that can occur in shallow bays that eelgrass commonly inhabit. For example, extensive high temperature events have been observed in Scotian Shelf eelgrass meadows with temperatures exceeding 23 °C (Wong et al. 2013, 2020; Krumhansl et al. 2020). Such temperatures can cause reduced growth and performance, with acute shoot mortality occurring at 30 °C or higher (Nejrup and Pedersen 2008; Marín‐Guirao et al. 2019). With marine heatwaves recently appearing on both the Pacific (Kintisch 2015) and Atlantic coasts (Mills et al. 2013) of Canada, it is likely that extreme temperature events will continue to occur with climate change.
6.5. Eelgrass wasting disease
While eelgrass wasting disease is not a human impact, we include it here because of its potential to affect eelgrass ecosystems and be exacerbated by some of the anthropogenic drivers described above. Eelgrass wasting disease is caused by Labyrinthula zosterae, a marine slime mold-like protist that infects eelgrass (Muehlstein et al. 1991). This opportunistic pathogen degrades cell walls and destroys the cytoplasm, thus breaking down chloroplasts and creating dark, necrotic lesions on the leaves (Muehlstein 1992). The lack of chloroplasts reduces photosynthetic efficiency near the necrotic regions, which makes the plant more susceptible to dying (Ralph and Short 2002).
Wasting disease in eelgrass is suspected to have been the cause of a massive trans-Atlantic eelgrass die-off in the 1930s (Muehlstein et al. 1991; Sullivan et al. 2013), which is the most catastrophic eelgrass die-off on record (Muehlstein 1989). Eelgrass shoot length, meadow density, and low-light conditions may be drivers for more extreme wasting disease events, and diseased leaves produce more phenolic acids (Groner et al. 2016; Dawkins et al. 2018), which could be a stress response (McKone and Tanner 2009). Additionally, changes in temperature may exacerbate the effects of wasting disease since higher temperatures can lead to increased growth rates of L. zosterae (Dawkins et al. 2018). However, higher temperatures coupled with lower salinity may reduce wasting disease activity and aid with eelgrass recovery (Burdick et al. 1993; Brakel et al. 2019).
While eelgrass wasting disease has not been studied extensively in Canada, it is widespread from southern California to Alaska, including the Northeastern Pacific (Groner et al. 2016; Dawkins et al. 2018), and it has been historically documented in the Northwestern Atlantic (Cottam and Munro 1954; Short et al. 1986). Preliminary studies show that wasting disease is present along the coast of British Columbia and varies spatiotemporally in prevalence and severity (O.J. Graham, personal communication, 2020). However, eelgrass meadow decline has not yet been directly associated with wasting disease outbreaks in Canada. As seawater temperature continues to rise, there may be a detrimental effect of eelgrass wasting disease on eelgrass ecosystems along the Canadian coast.
7. Implications for management and conservation
7.1. Current eelgrass management and conservation in Canada
The management context of eelgrass ecosystems in Canada is complex. Different governing bodies have oversight over different parts of the depth-distribution of eelgrass along the coast. Addressing the use and protection of eelgrass meadows varies from being a predominantly federal responsibility in much of Canada for the areas that lie below the mean low-tide mark to variously including provincial, territorial, and even municipal roles for meadow sections above this threshold. For example, in some regions (e.g., Newfoundland and Labrador) eelgrass meadows are only found subtidally, placing them exclusively within federal purview. In contrast, British Columbia has large extensive eelgrass meadows in both intertidal and shallow subtidal areas, thus various protective measures spanning governments at federal, provincial, territorial, and municipal levels may apply. Many other provinces and territories have components of all these elements.
Further complicating eelgrass management in Canada are conflicting authorities even within bureaucratic levels. Federally, the authority to manage eelgrass meadows predominantly falls under the provisions of the Fisheries Act. The Act—first enacted in 1868—is one of the strongest pieces of environmental legislation in the world and is the responsibility of DFO, through its No Net Loss policy. However, in many bioregions, eelgrass management in specific habitats (e.g., Boundary Bay, British Columbia) also involves the Migratory Bird Convention Act (Environment Canada) because of the known importance of eelgrass habitats for taxa such as commercial and forage fishes and migratory birds. Similar complications are common in all Canadian marine bioregions.
Since 2009, the Government of Canada considers eelgrass to be an ecologically significant species (DFO 2009a). This means that the ecological consequences of perturbing eelgrass are deemed to be substantially greater than those of perturbing most other species associated with the marine coastal zone (DFO 2009a, 2011). Although this designation does not ensure that all eelgrass habitats receive protection from all threats, it does provide a strong basis for enhanced caution when considering such threats within a management context (DFO 2011). The No Net Loss policy of the Fisheries Act suggests that any loss of eelgrass habitat needs to be offset by creation of similar habitat elsewhere (Minns et al. 2011). However, eelgrass habitat replacement is difficult to achieve when large meadows are impacted; therefore, avoidance or mitigation is often a preferred option. For example, building on Canada’s success at meeting its commitment to protect 10% of coastal and marine areas by 2020 (Aichi Target 11), the newly mandated goal of increasing protection to 25% by 2025 and to 30% by 2030 (Minister of Fisheries, Oceans and the Canadian Coast Guard Mandate Letter 2019), provides additional incentives and opportunities to protect eelgrass ecosystems through Marine Protected Area (MPA) Networks and other area-based conservation planning initiatives (e.g., DFO 2017, 2019). Currently, there are only a few, generally small, coastal MPAs in Atlantic Canada that harbour eelgrass, such as Basin Head MPA in Prince Edward Island (Fisheries and Oceans Canada 2020). In contrast, eelgrass on the Pacific Coast is present in many small provincial and a few federally protected areas. Mapping data suggest that ∼31% of eelgrass meadows in the Northern Shelf Bioregion are located within existing protected areas albeit with varying levels of protection, but little information is available on specific eelgrass management (E. Rubidge, unpublished data). While representation of eelgrass in MPA networks alone will not ensure complete protection, setting clear targets for eelgrass inclusion in network planning should produce significant conservation outcomes. Similarly, the inclusion of eelgrass in federal or provincial coastal protected areas and wildlife management areas could be a significant contributor to the protection of eelgrass ecosystems.
7.2. Integrating science and management
Strong seasonal changes in eelgrass growth, survival and canopy structure have important implications for the timing of management and conservation strategies—e.g., restoration efforts or environmental impact assessments (see Box 1). Similarly, the strong influence of environmental conditions and anthropogenic impacts on local and regional scales highlights the importance of correctly guiding the locations of management and conservation actions—e.g., the placement of marine use zones, pollution interventions, and eelgrass restoration activities (see Section 6).
Box 1. Five science-based rules of thumb for eelgrass management across Canada and how they link back to specific sections of this synthesis paper.
Rules of Thumb for Eelgrass Management
1.
Consider the role of eelgrass habitats to be managed in a landscape context, i.e., the distribution of meadows and patches and their connectivity, which differs spatially and seasonally among Pacific, Atlantic, and Arctic coasts (Sections 4 and 5, DFO 2009a).
2.
3.
Consider the species associated with eelgrass habitats, either year-round or during parts of the year, particularly those that are of commercial or conservation interest or support coastal food webs (Section 5).
4.
Take into account regionally relevant human impacts and concerns regarding cumulative and chronic versus acute effects (Section 6, DFO 2011).
5.
Monitoring plans associated with human impacts should include baseline conditions. Further, to be effective, they must incorporate seasonal elements, especially including seasons of peak eelgrass growth and production—when plants and associated fauna will be most active and apparent (Sections 4–7).
A major challenge when managing eelgrass habitats across Canada is that guidance that applies to one region, may not be suitable in another, given regional variation in environmental conditions, human activities, and eelgrass characteristics we have outlined throughout this paper. However, despite differences in eelgrass-dominated landscapes and ecosystems among Canada’s coasts, there are several general principles that may help to guide regional or local management regardless of the specifics of regional geography. Along with the general suggestion of preventing the harmful alteration, disruption, or destruction (HADD) of eelgrass habitats (Section 6, DFO 2011; Murphy et al. 2019), we offer five science-based rules of thumb with which managers could engage regional eelgrass scientists along the Pacific, Atlantic, and Arctic coasts to identify ways forward when managing eelgrass ecosystems (Box 1).
In addition to these general rules of thumb, more detailed information to advise various regulatory authorities on eelgrass management and conservation can be provided by multiple sources, including governments, academia, First Nations, traditional ecological knowledge holders, coastal stakeholders, and nonprofit environmental and stewardship groups. Ideally, these sources of information would be integrated and combined to derive the most meaningful management targets and conservation strategies within and across bioregions. For example, Wilson et al. (2019) used remote sensing data in combination with substrate mapping, local ecological knowledge, and site-specific information from government and nongovernment sources to produce integrated maps of eelgrass and macroalgae habitat on Nova Scotia’s southern shore. However, while eelgrass mapping is more comprehensive along the Pacific coast (see Fig. 1, BCMCA 2011), basic eelgrass distribution mapping is lacking in many parts of Atlantic Canada prohibiting quantitative habitat assessments to achieve management targets. Also, many eelgrass studies, surveys and assessments across Canada are done in isolation; therefore, even basic comparisons of trends in eelgrass bed extent and condition (e.g., Fig. 2) are difficult at this time. Thus, operationalizing existing scientific knowledge of eelgrass ecosystems into standardized integrated management advice across Canada remains a challenge.
Many of the concepts needed to operationalize eelgrass ecosystem and human impact metrics (Sections 4–6) into management guidance have been previously explored in a broader context. For example, the productive capacity of eelgrass meadows can be used to assess their importance as fish habitat (Minns et al. 2011). Such explorations provide useful starting points from which to evaluate management practice affecting eelgrass habitats. Despite substantial knowledge gaps, which we have highlighted, our general understanding of eelgrass dynamics, ecosystem value, and linkages up the food chain—e.g., to invertebrate and fish productivity—have been better studied for eelgrass than most other marine habitats in Canada.
Standardizing all eelgrass monitoring efforts across Canada is not appropriate or possible for all metrics and community components given regional differences in eelgrass meadows, associated communities, environmental conditions, and human impacts (see Sections 3–6). The most substantive methods to attempt to achieve standardization are those that provide metrics to map and measure the spatial distribution of eelgrass meadows, habitat patches, and plants in the coastal environment. Furthermore, a consistent understanding of environmental and human impact gradients and how they influence eelgrass ecosystems within and across bioregions is key to determining appropriate approaches to eelgrass management and protection. Lastly, incorporating climate change projections into management decisions is essential, whether it will act to limit future eelgrass growth and survival in shallow bays along the Atlantic and Pacific coasts or allow eelgrass distribution to expand in the Arctic (see Section 6).
The management of human impacts on eelgrass habitats in Canada requires a national, regional, and local context. Although the list of negative human impacts applies to all coasts and bioregions (Section 6), their management needs to be adapted to regional or local circumstances. On a local scale, stressors affecting a specific eelgrass meadow depend on the dominant and cumulative human impacts within a region as well as environmental background conditions (Murphy et al. 2019). The resilience of eelgrass ecosystems to stressors also depends on season- and region-specific growth factors and phenology (Wu et al. 2017), which need to be considered in management strategies. For example, coastal construction and resulting erosion or run-off effects could be limited to times when eelgrass is least likely to be deleteriously affected. In the Arctic, avoiding stress during peak growth periods may be critically important as eelgrass grow at the limit of their physiological tolerance, compared with the Pacific and Atlantic coasts, where repeated stressors may be relatively more substantive factors.
The conservation of eelgrass habitats also requires a multi-level approach across Canada. For example, large extensive eelgrass meadows, such as those associated with the Fraser River delta and the Skeena River estuary in British Columbia, are obvious targets for protection. However, small patchy eelgrass meadows, which predominate along the Pacific coast and Atlantic coasts of Nova Scotia, and Newfoundland, also form significant marine habitat components. Habitat connectivity among eelgrass meadows as well as among eelgrass, kelp, rockweed and other marine habitats (e.g., Olson et al. 2019) should also be considered in MPA networks and other spatial conservation plans on regional scales.
Links from eelgrass habitat management and conservation to commercial fisheries are important considerations across all regions, whether directly or indirectly supported by eelgrass habitats (e.g., Tupper and Boutilier 1995; Gotceitas et al. 1997; Cote et al. 2013). Some human impacts could be easily prevented through awareness or compliance measures, such as the loss or damage of eelgrass due to fishing or aquaculture practices (e.g., storage of fishing gear or shellfish bags on eelgrass meadows). The link from eelgrass meadows to other important ecosystem services, such as shoreline protection, nutrient cycling, or blue carbon storage, could also help to promote eelgrass management and conservation across Canada. Many coastal residents, visitors, resource users, or other stakeholders may not know what eelgrass is or what important roles eelgrass habitats play for marine ecosystems and human societies. Here, public awareness and education programs across Canada’s marine bioregions could enhance individual and common knowledge of eelgrass, its values and threats (e.g., Guest et al. 2015). Canada’s specific challenges integrating science and management for eelgrass conservation, lie in the country’s vast coastline, which encompasses wide environmental and human use gradients and varying jurisdictional complexity in foreshore, intertidal, and subtidal areas. Governance complexity and a wide range of environmental variation provide a unique opportunity for a large-scale coordinated research effort linked with management questions that could inform global management of eelgrass. Research efforts in understudied regions in northern latitudes and the Arctic can contribute to global gaps in our understanding of eelgrass ecology, and have particular significance to management of this important species under global change.
8. Conclusions
Our synthesis provides an overview and summary of the growing body of eelgrass research in Canada over the past decades. It highlights the commonalities as well as differences among eelgrass ecosystems from coast to coast to coast, including wide gradients in environmental conditions and human influences, which pose specific management and conservation challenges. Our synthesis also stresses the interconnectedness of environmental and human influences with eelgrass habitat structure, ecosystem functions, and food-web dynamics on local and regional scales requiring a holistic and integrated approach to coastal ecosystem conservation and management on different spatial scales and government levels. The issues and challenges we have highlighted can be informative towards developing regional and global strategies for the assessment and protection of seagrass ecosystems or other vegetated habitats across many countries and climate zones worldwide. Our work could also act as a template for similar syntheses of seagrass ecosystems or other habitat types in other parts of the world to foster the comparability of habitat status, trends, threats, and management strategies. Finally, we highlight many changes in environmental and human influences across Canada’s bioregions in the context of climate and global change. These influences are expected to continue to affect and alter eelgrass distribution and ecosystem functioning in coming decades worldwide.
Acknowledgements
Financial support was provided by the Natural Sciences and Engineering Research Council (NSERC) of Canada’s Canadian Healthy Oceans Network (CHONe II) and its Partners: Fisheries and Oceans Canada and INREST (representing the Port of Sept-Îles and City of Sept-Îles). SJB also acknowledges funding through the Liber Ero Postdoctoral Fellowship, JCD through NSERC (CGSD3-518641-2018), and HKL through NSERC (RGPIN-2014-04491).
References
Amundrud SL, Srivastava DS, and O’Connor MI. 2015. Indirect effects of predators control herbivore richness and abundance in a benthic eelgrass (Zostera marina) mesograzer community. Journal of Animal Ecology, 84: 1092–1102.
Baker DGL, Eddy TD, McIver R, Schmidt AL, Thériault M-H, Boudreau M, et al. 2016. Comparative analysis of different survey methods for monitoring fish assemblages in coastal habitats. PeerJ, 4: e1832.
Barbier EB, Hacker SD, Kennedy C, Koch EW, Stier AC, and Silliman BR. 2011. The value of estuarine and coastal ecosystem services. Ecological Monographs, 81(2): 169–193.
Barrell J, Grant J, Hanson A, and Mahoney M. 2015. Evaluating the complementarity of acoustic and satellite remote sensing for seagrass landscape mapping. International Journal of Remote Sensing, 36(16): 4069–4094.
Beca-Carretero P, Olesen B, Marbà N, and Krause-Jensen D. 2018. Response to experimental warming in northern eelgrass populations: comparison across a range of temperature adaptations. Marine Ecology Progress Series, 589: 59–72.
Bednaršek N, Feely RA, Beck MW, Alin SR, Siedlecki SA, Calosi P, et al. 2020. Exoskeleton dissolution with mechanoreceptor damage in larval Dungeness crab related to severity of present-day ocean acidification vertical gradients. Science of the Total Environment, 716: 136610.
Berkenbusch K, Rowden AA, and Myers TE. 2007. Interactions between seagrasses and burrowing ghost shrimps and their influence on infaunal assemblages. Journal of Experimental Marine Biology and Ecology, 341(1): 70–84.
Biebl R, and McRoy CP. 1971. Plasmatic resistance and rate of respiration and photosynthesis of Zostera marina at different salinities and temperatures. Marine Biology, 8: 48–56.
Blok S, Olesen B, and Krause-Jensen D. 2018. Life history events of eelgrass Zostera marina L. populations across gradients of latitude and temperature. Marine Ecology Progress Series, 590: 79–93.
Boese BL, Alayan KE, Gooch EF, and Robbins BD. 2003. Desiccation index: a measure of damage caused by adverse aerial exposure on intertidal eelgrass (Zostera marina) in an Oregon (USA) estuary. Aquatic Botany, 76(4): 329–337.
Bonsal BR, Peters DL, Seglenieks F, Rivera A, and Berg A. 2019. Changes in freshwater availability across Canada; Chapter 6. In Canada’s changing climate report. Edited by E Bush and DS Lemmen. Government of Canada, Ottawa, Ontario. pp. 261–342.
Bradbury IR, Laurel BJ, Robichaud DR, Rose GA, Snelgrove PVR, Gregory RS, et al. 2008. Discrete spatial dynamics in a marine broadcast spawner: re-evaluating scales of connectivity and habitat associations in Atlantic cod (Gadus morhua) in coastal Newfoundland. Fisheries Research, 91: 299–309.
Brakel J, Jakobsson-Thor S, Bockelmann A, and Thorsten BH. 2019. Modulation of the eelgrass—Labyrinthula zosterae interaction under predicted ocean warming, salinity change and light limitation. Frontiers in Marine Science, 6: 268.
Bricker SB, Longstaff B, Dennison W, Jones A, Boicourt K, Wicks C, et al. 2008. Effects of nutrient enrichment in the nation’s estuaries: a decade of change. Harmful Algae, 8(1): 21–32.
Bristow LA, Mohr W, Ahmerkamp S, and Kuypers MMM. 2017. Nutrients that limit growth in the ocean. Current Biology, 27: R474–R478.
British Columbia Marine Conservation Analysis Project Team (BCMCA). 2011. Marine atlas of Pacific Canada: a product of the British Columbia marine conservation analysis [online]: Available from bcmca.ca.
Brock TD. 1981. Calculating solar radiation for ecological studies. Ecological Modelling, 14(1–2): 1–19.
Bugden G, Jiang Y, MacQuarrie KTB, Crane CJ, and Raymond BG. 2014. Nitrogen loading criteria for estuaries in Prince Edward Island. Canadian Science Advisory Secretariat Science Advisory Report 3066. Fisheries and Oceans Canada.
Burd BJ, Barnes PAG, Wright CA, and Thomson RE. 2008. A review of subtidal benthic habitats and invertebrate biota of the Strait of Georgia, British Columbia. Marine Environmental Research, 66: S3–S38.
Burdick DM, Short FT, and Wolf J. 1993. An index to assess and monitor the progression of wasting disease in eelgrass Zostera marina. Marine Ecology Progress Series, 94: 83–90.
Burke M, Dennison W, and Moore K. 1996. Non-structural carbohydrate reserves of eelgrass Zostera marina. Marine Ecology Progress Series, 137: 195–201.
Bush E, and Lemmen DS. 2019. Canada’s changing climate report. Government of Canada, Ottawa, Ontario. 444 p.
Carlson PR Jr, Yarbo LA, Kaufman KA, and Mattson RA. 2010. Vulnerability and resilience of seagrasses to hurricane and runoff impacts along Florida’s west coast. Hydriobiologia, 649: 39–53.
Carman MR, Colarusso PD, Neckles HA, Bologna P, Caines S, Davidson JDP, et al. 2019. Biogeographical patterns of tunicates utilizing eelgrass as substrate in the western North Atlantic between 39° and 47° north latitude (New Jersey to Newfoundland). Management of Biological Invasions, 10(4): 602–616.
Chalifour L, Scott D, MacDuffee M, Iacarella J, Martin T, and Baum J. 2019. Habitat use by juvenile salmon, other migratory fish, and resident fish species underscores the importance of estuarine habitat mosaics. Marine Ecology Progress Series, 625: 145–162.
Clausen K, Krause-Jensen D, Olesen B, and Marbà N. 2014. Seasonality of eelgrass biomass across gradients in temperature and latitude. Marine Ecology Progress Series, 506: 71–85.
Coffin MRS, Courtenay SC, Knysh KM, Pater CC, and van den Heuvel MR. 2018. Impacts of hypoxia on estuarine macroinvertebrate assemblages across a regional nutrient gradient. FACETS, 3: 23–44.
Coll M, Schmidt A, Romanuk T, and Lotze HK. 2011. Food-web structure and seagrass communities across different spatial scales and human impacts. PLoS ONE, 6: e22591.
Copeman LA, Parrish CC, Gregory RS, Jamieson RE, Wells J, and Whiticar MJ. 2009. Fatty acid biomarkers in coldwater eelgrass meadows: elevated terrestrial input to the food web of age-0 Atlantic cod Gadus morhua. Marine Ecology Progress Series, 386: 237–251.
Cote D, Gregory RS, Morris CJ, Newton BH, and Schneider DC. 2013. Elevated habitat quality reduces variance in fish community composition. Journal of Experimental Marine Biology and Ecology, 440: 22–28.
Cottam C, and Munro DA. 1954. Eelgrass status and environmental relations. Journal of Wildlife Management, 18: 449–460.
Cullain N, McIver R, Schmidt AL, and Lotze HK. 2018a. Spatial variation of macroinfaunal communities associated with Zostera marina bed across three biogeographic regions in Atlantic Canada. Estuaries and Coasts, 41: 1381–1396.
Cullain N, McIver R, Schmidt AL, Milewski I, and Lotze HK. 2018b. Potential impacts of finfish aquaculture on eelgrass (Zostera marina) beds and possible monitoring metrics for management: a case study in Atlantic Canada. PeerJ, 6: e5630.
Cummins PF, and Masson D. 2014. Climatic variability and trends in the surface waters of coastal British Columbia. Progress in Oceanography, 120: 279–290.
Curry CL, Islam SU, Zwiers FW, and Déry SJ. 2019. Atmospheric rivers increase future flood risk in western Canada’s largest Pacific river. Geophysical Research Letters, 46(3): 1651–1661.
Dalley KL, Gregory RS, Morris CJ, and Cote D. 2017. Seabed habitat determines fish and macroinvertebrate community associations in a subarctic marine coastal nursery. Transactions of the American Fisheries Society, 146(6): 1115–1125.
Daru BH, Holt BG, Lessard J-P, Yessoufou K, and Davies TJ. 2017. Phylogenetic regionalization of marine plants reveals close evolutionary affinities among disjunct temperate assemblages. Biological Conservation, 213: 351–356.
Dawkins PD, Eisenlord ME, Yoshioka RM, Fiorenza E, Fruchter S, Giammona F, et al. 2018. Environment, dosage, and pathogen isolate moderate virulence in eelgrass wasting disease. Diseases of Aquatic Organisms, 130(1): 51–63.
de los Santos CB, Krause-Jensen D, Alcoverro T, Marba N, Duarte CM, vaan Katwijk MM, et al. 2019. Recent trend reversal for declining European seagrass meadows. Nature Communications, 10: 3356.
Dennison WC, and Alberte RS. 1982. Photosynthetic responses of Zostera marina L. (Eelgrass) to in situ manipulations of light intensity. Oecologia, 55(2): 137–144.
Department of Fisheries and Oceans Canada (DFO). 2007. Guidance document on identifying conservation priorities and phrasing conservation objectives for large ocean management areas. Canadian Science Advisory Secretariat Science Advisory Report 2007/010. Department of Fisheries and Oceans Canada.
Department of Fisheries and Oceans Canada (DFO). 2009a. Does eelgrass (Zostera marina) meet the criteria as an ecologically and significant species? Canadian Science Advisory Secretariat Science Advisory Report 2009/018. Department of Fisheries and Oceans Canada.
Department of Fisheries and Oceans Canada (DFO). 2009b. Development of a framework and principles for the biogeographic classification of Canadian marine areas. Canadian Science Advisory Secretariat Science Advisory Report 2009/056. Department of Fisheries and Oceans Canada.
Department of Fisheries and Oceans Canada (DFO). 2011. Definitions of harmful alteration, disruption or destruction (HADD) of habitat provided by eelgrass (Zostera marina). Canadian Science Advisory Secretariat Science Advisory Report 2011/058. Department of Fisheries and Oceans Canada.
Department of Fisheries and Oceans Canada (DFO). 2016. Federal marine bioregions [online]: Available from open.canada.ca/data/en/dataset/23eb8b56-dac8-4efc-be7c-b8fa11ba62e9.
Department of Fisheries and Oceans Canada (DFO). 2017. Oceanographic conditions in the Atlantic zone in 2017. Canadian Science Advisory Secretariat Science Advisory Report 2018/039. Department of Fisheries and Oceans Canada.
Department of Fisheries and Oceans Canada (DFO). 2019. Design strategies for the Northern Shelf Bioregional Marine Protected Area Network. Canadian Science Advisory Secretariat Science Advisory Report 2019/026. Department of Fisheries and Oceans Canada.
Díaz S, and Cabido M. 2001. Vive la différence: plant functional diversity matters to ecosystem processes. Trends in Ecology & Evolution, 16: 646–655.
Duarte CM. 1991. Seagrass depth limits. Aquatic Botany, 40: 363–377.
Duffy JE, Reynolds PL, Boström C, Coyer JA, Cusson M, Donadi S, et al. 2015. Biodiversity mediates top-down control in eelgrass ecosystems: a global comparative-experimental approach. Ecology Letters, 18(7): 696–705.
Durance C. 2002. Methods for mapping and monitoring eelgrass habitats in British Columbia. Canadian Wildlife Service, Environment Canada.
Egea LG, Jimenez-Ramos R, Hernandez I, Bouma TJ, and Brun FG. 2018. Effects of ocean acidification and hydrodynamic conditions on carbon metabolism and dissolved organic carbon (DOC) fluxes in seagrass populations. PLoS ONE, 13: e0192402.
Erftemeijer PLA, and Lewis RRR. 2006. Environmental impacts of dredging on seagrasses: a review. Marine Pollution Bulletin, 52: 1553–1572.
Eriander L, Laas K, Bergström P, Gipperth L, and Moksnes PO. 2017. The effects of small-scale coastal development on the eelgrass (Zostera marina L.) distribution along the Swedish west coast—ecological impact and legal challenges. Ocean & Coastal Management, 148: 182–194.
ESRI. 2019. World Country Boundaries 2019 [basemap] [online]: Available from services.arcgis.com/P3ePLMYs2RVChkJx/arcgis/rest/services/WOR_Boundaries_2019/FeatureServer.
Fisheries and Oceans Canada. 2020. Marine Protected Areas across Canada [online]: Available from dfo-mpo.gc.ca/oceans/mpa-zpm/index-eng.html.
Foreman MGG, Pal B, and Merryfield WJ. 2011. Trends in upwelling and downwelling winds along the British Columbia shelf. Journal of Geophysical Research: Oceans, 116: C10023.
Gale KS, Frid A, Lee L, McCarthy J-B, Robb C, Rubidge E, et al. 2018. A framework for identification of ecological conservation priorities for Marine Protected Area network design and its application in the Northern Shelf Bioregion. Canadian Science Advisory Secretariat Science Advisory Report. Fisheries and Oceans Canada.
Gallegos CL. 2001. Calculating optical water quality targets to restore and protect submersed aquatic vegetation: overcoming problems in partitioning the diffuse attenuation coefficient for photosynthetically active radiation. Estuaries, 24(3): 381–397.
Gallegos CL, and Moore KA. 2000. Factors contributing to water-column light attenuation. In Chesapeake Bay submerged aquatic vegetation water quality and habitat-based requirements and restoration targets: a second technical synthesis. Edited by RA Batiuk, P Bergstrom, WM Kemp, E Koch, L Murray, JC Stevenson, et al. U.S. Environmental Protection Agency, Chesapeake Bay Program, Annapolis, Maryland. pp. 16–27.
Garbary DJ, Miller AG, Williams J, and Seymour NR. 2014. Drastic decline of an extensive eelgrass bed in Nova Scotia due to the activity of the invasive green crab (Carcinus maenas). Marine Biology, 161: 3–15.
Garrett CJR, and Loucks H. 1976. Upwelling along the Yarmouth shore of Nova Scotia. Journal of the Fisheries Research Board of Canada, 33(1): 116–117.
Gattuso J-P, Gentili B, Duarte CM, Kleypas JA, Middelburg JJ, and Antoine D. 2006. Light availability in the coastal ocean: impact on the distribution of benthic photosynthetic organisms and their contribution to primary production. Biogeosciences, 3: 489–513.
Gillespie GE, Norgard TC, Anderson ED, Haggarty DR, and Phillips AC. 2015. Distribution and biological characteristics of European Green Crab, Carcinus maenas, in British Columbia, 2006–2013. Canadian Technical Report of Fisheries and Aquatic Sciences 3120.
GlobColour Project. 2019 [online]: Available from globcolour.info.
Gorman AM, Gregory RS, and Schneider DC. 2009. Eelgrass patch size and proximity to the patch edge affect predation risk of recently settled age 0 cod (Gadus). Journal of Experimental Marine Biology and Ecology, 371: 1–9.
Gotceitas V, Fraser S, and Brown JA. 1997. Use of eelgrass beds (Zostera marina) by juvenile Atlantic cod (Gadus morhua). Canadian Journal of Fisheries and Aquatic Sciences, 54: 1306–1319.
Government of Nunavut. 2010. Nunavut Coastal Resource Inventory [online]: Available from gov.nu.ca/environment/information/nunavut-coastal-resource-inventory.
Govers LL, de Brouwer JH, Suykerbuyk W, Bouma TJ, Lamers LP, Smolders AJ, et al. 2014. Toxic effects of increased sediment nutrient and organic matter loading on the seagrass Zostera noltii. Aquatic Toxicology, 155: 253–260.
Granskog MA, Kuzyk ZZA, Azetsu-Scott K, and Macdonald RW. 2011. Distributions of runoff, sea-ice melt and brine using δ18O and salinity data—a new view on freshwater cycling in Hudson Bay. Journal of Marine Systems, 88(3): 362–374.
Green EP, and Short FT. 2003. World atlas of seagrasses. UNEP-WCMC.
Greene CH, and Pershing AJ. 2007. Climate drives sea change. Science, 315(5815): 1084–1085.
Gregory RS, Dalley KL, Newton BH, Sargent PS, and Cooke ELL. 2019. Relative strength of three cohorts (2015-17) of Atlantic cod, from nearshore surveys of demersal age 0 and 1 juveniles in Newman Sound, Bonavista Bay. Canadian Science Advisory Secretariat Research Document 2019/012.
Groner ML, Burge CA, Kim CJS, Rees E, Van Alstyne KL, Yang S, et al. 2016. Plant characteristics associated with widespread variation in eelgrass wasting disease. Diseases of Aquatic Organisms, 118(2): 159–168.
Guest H, Lotze HM, and Wallace D. 2015. Youth and the sea: ocean literacy in Nova Scotia, Canada. Marine Policy, 58: 98–107.
Guidetti P. 2000. Differences among fish assemblages associated with nearshore Posidonia oceanica seagrass beds, rocky–algal reefs and unvegetated sand habitats in the Adriatic Sea. Estuarine, Coastal and Shelf Science, 50(4): 515–529.
Harrison PG. 1987. Natural expansion and experimental manipulation of seagrass (Zostera spp.) abundance and the response of infaunal invertebrates. Estuarine, Coastal and Shelf Science, 24: 799–812.
Harrison PG, and Bigley RE. 1982. The recent introduction of the seagrass Zostera japonica Aschers. and Graebn. to the Pacific coast of North America. Canadian Journal of Fisheries and Aquatic Sciences, 39: 1642–1648.
Hauxwell J, Cebrián J, and Valiela I. 2003. Eelgrass Zostera marina loss in temperate estuaries: relationship to land-derived nitrogen loads and effect of light limitation imposed by algae. Marine Ecology Progress Series, 247: 59–73.
Heck KL, Carruthers TJB, Duarte CM, Hughes AR, Kendrick G, Orth RJ, et al. 2008. Trophic transfers from seagrass meadows subsidize diverse marine and terrestrial consumers. Ecosystems, 11(7): 1198–1210.
Hemminga MA, and Duarte CM. 2008. Seagrass ecology. Cambridge University Press, Cambridge, UK.
Hessing-Lewis ML, and Hacker SD. 2013. Upwelling-influence, macroalgal blooms, and seagrass production; temporal trends from latitudinal and local scales in northeast Pacific estuaries. Limnology and Oceanography, 58: 1103–1112.
Hessing-Lewis ML, Rechsteiner EU, Hughes BB, Tinker TM, Monteith ZL, Olson AM, et al. 2018. Ecosystem features determine seagrass community response to sea otter foraging. Marine Pollution Bulletin, 134: 134–144.
Hitchcock JK, Courtenay SC, Coffin MRS, Pater CC, and van den Heuvel MR. 2017. Eelgrass bed structure, leaf nutrient, and leaf isotope responses to natural and anthropogenic gradients in estuaries of the Southern Gulf of St. Lawrence, Canada. Estuaries and Coasts, 40(6): 1653–1665.
Hooper DU, Chapin III FS, and Ewel JJ. 2005. Effects of biodiversity on ecosystem functioning: a consensus of current knowledge. Ecological Monographs, 75: 3–35.
Howard BR, Francis FT, Côté IM, and Therriault TW. 2019. Habitat alteration by invasive European green crab (Carcinus maenas) causes eelgrass loss in British Columbia, Canada. Biological Invasions, 21: 3607–3618.
Huang AC, Essak M, and O’Connor MI. 2015. Top–down control by great blue herons Ardea herodias regulates seagrass-associated epifauna. Oikos, 124(11): 1492–1501.
Hughes A, Bando K, Rodriguez L, and Williams S. 2004. Relative effects of grazers and nutrients on seagrasses: a meta-analysis approach. Marine Ecology Progress Series, 282: 87–99.
Iacarella JC, Adamczyk E, Bowen D, Chalifour L, Eger A, Heath W, et al. 2018. Anthropogenic disturbance homogenizes seagrass fish communities. Global Change Biology, 24(5): 1904–1918.
Ings DW, Gregory RS, and Schneider DC. 2008. Episodic downwelling predicts recruitment of Atlantic cod, Greenland cod and white hake to Newfoundland coastal waters. Journal of Marine Research, 66: 529–561.
Jarvis JC, Moore KA, and Kenworthy WJ. 2012. Characterization and ecological implication of eelgrass life history strategies near the species’ southern limit in the western North Atlantic. Marine Ecology Progress Series, 444: 43–56.
Joseph V, Locke A, and Godin J-GJ. 2006. Spatial distribution of fishes and decapods in eelgrass (Zostera marina) and sandy habitats of a New Brunswick estuary, eastern Canada. Aquatic Ecology, 40: 111–123.
Keddy CJ, and Patriquin DG. 1978. An annual form of eelgrass in Nova Scotia. Aquatic Botany, 5: 163–170.
Kelly JJ, Orr D, and Takekawa JY. 2019. Quantification of damage to eelgrass (Zostera marina) beds and evidence-based management strategies for boats anchoring in San Francisco Bay. Environmental Management, 64: 20–26.
Kim SH, Kim J-H, Park SR, and Lee K-S. 2014. Annual and perennial life history strategies of Zostera marina populations under different light regimes. Marine Ecology Progress Series, 509: 1–13.
Kintisch E. 2015. ‘The Blob’ invades Pacific, flummoxing climate experts. Science, 348: 17–18.
Kirk JT. 2010. Light and photosynthesis in aquatic ecosystems. 3rd edition. Cambridge University Press, New York, New York.
Klassen G, and Locke A. 2007. A biological synopsis of the European Green Crab, Carcinus maenas. Canadian Manuscript Report of Fisheries and Aquatic Sciences No. 2818. vii + 75 p.
Knight NS, Prentice C, Tseng M, and O’Connor MI. 2015. A comparison of epifaunal invertebrate communities in native eelgrass Zostera marina and non-native Zostera japonica at Tsawwassen, BC. Marine Biology Research, 11: 564–571.
Koch M, Bowes G, Ross C, and Zhang XH. 2013. Climate change and ocean acidification effects on seagrasses and marine macroalgae. Global Change Biology, 19(1): 103–132.
Krumhansl K, Dowd M, and Wong MC. 2020. A characterization of the physical environment at seagrass sites along the Atlantic coast of Nova Scotia. Canadian Technical Report of Fisheries and Aquatic Sciences 3361. Fisheries and Oceans Canada. iv + 213 p.
Lalumière R, Messier D, Fournier J-J, and McRoy P. 1994. Eelgrass meadows in a low arctic environment, the northeast coast of James Bay, Québec. Aquatic Botany, 47: 303–315.
Laurel BJ, Gregory RS, and Brown JA. 2003a. Settlement and distribution of age 0 juvenile cod, Gadus morhua and Gadus ogac, following a large-scale habitat manipulation. Marine Ecology Progress Series, 262: 241–252.
Laurel BJ, Gregory RS, and Brown JA. 2003b. Predator distribution and habitat patch area determine predation rates on Age-0 juvenile cod Gadus spp. Marine Ecology Progress Series, 251: 245–254.
Laurel BJ, Cote D, Gregory RS, Rogers L, Knutsen H, and Olsen EM. 2017. Recruitment signals in juvenile cod surveys depend on thermal growth conditions. Canadian Journal of Fisheries and Aquatic Sciences, 74: 511–523.
Lee K-S, Park SR, and Kim YK. 2007. Effects of irradiance, temperature, and nutrients on growth dynamics of seagrasses: a review. Journal of Experimental Marine Biology and Ecology, 350(1–2): 144–175.
Lee SY, Dunn RJK, Young RA, Connolly RM, Dale PER, Dehayr R, et al. 2006. Impact of urbanization on coastal wetland structure and function. Austral Ecology, 31: 149–163.
Lefcheck JS, Orth RJ, Dennison WC, Wilcox DJ, Murphy RR, Keisman J, et al. 2018. Long-term nutrient reductions lead to the unprecedented recovery of a temperate coastal region. Proceedings of the National Academy of Sciences of the United States of America, 115(14): 3658–3662.
Linehan JE, Gregory RS, and Schneider DC. 2001. Predation risk of age-0 cod Gadus relative to depth and substrate in coastal waters. Journal of Experimental Marine Biology and Ecology, 263: 25–44.
Lins DM, de Marco P Jr, Andrade AFA, and Rocha RM. 2018. Predicting global ascidian invasions. Diversity and Distributions, 24: 692–704.
Long Z, Perrie W, Chassé J, Brickman D, Guo L, Drozdowski A, et al. 2016. Impacts of climate change in the Gulf of St. Lawrence. Atmosphere-Ocean, 54(3): 337–351.
Lotze HK, and Milewski I. 2004. Two centuries of multiple human impacts and successive changes in a North Atlantic food web. Ecological Applications, 14(5): 1428–1447.
Lotze HK, Lenihan HS, Bourque BJ, Bradbury RH, Cooke RG, Kay MC, et al. 2006. Depletion, degradation, and recovery potential of estuaries and coastal seas. Science, 312(5781): 1806–1809.
Lowen JB, and DiBacco C. 2017. Distributional changes in a guild of non-indigenous tunicates in the NW Atlantic under high-resolution climate projections. Marine Ecology Progress Series, 570: 173–186.
Mach ME, Wyllie-Echeverria S, and Chan KMA. 2014. Ecological effect of a nonnative seagrass spreading in the Northeast Pacific: a review of Zostera japonica. Ocean & Coastal Management, 102: 375–382.
Mach ME, Levings CD, and Chan KMA. 2017. Nonnative species in British Columbia eelgrass beds spread via shellfish aquaculture and stay for the mild climate. Estuaries and Coasts, 40(1): 187–199.
Mackas DL, and Harrison PJ. 1997. Nitrogenous nutrient sources and sinks in the Juan de Fuca Strait/Strait of Georgia/Puget Sound estuarine system: assessing the potential for eutrophication. Estuarine, Coastal and Shelf Science, 44(1): 1–21.
Malyshev A, and Quijón PA. 2011. Disruption of essential habitat by a coastal invader: new evidence of the effects of green crabs on eelgrass beds. ICES Journal of Marine Science, 68: 1852–1856.
Marbà N, and Duarte CM. 2010. Mediterranean warming triggers seagrass (Posidonia oceanica) shoot mortality. Global Change Biology, 16: 2366–2375.
Marín‐Guirao L, Entrambasaguas L, Ruiz JM, and Procaccini G. 2019. Heat-stress induced flowering can be a potential adaptive response to ocean warming for the iconic seagrass Posidonia oceanica. Molecular Ecology, 28(10): 2486–2501.
Marion SR, and Orth RJ. 2010. Factors influencing seedling establishment rates in Zostera marina and their implications for seagrass restoration. Restoration Ecology, 18(4): 549–559.
Marsh JA, Dennison WC, and Alberte RS. 1986. Effects of temperature on photosynthesis and respiration in eelgrass (Zostera marina L.). Journal of Experimental Marine Biology and Ecology, 101(3): 257–267.
Matheson K, McKenzie CH, Gregory RS, Robichaud DA, Bradbury IR, Snelgrove PVR, et al. 2016. Linking eelgrass decline and impacts on associated fish communities to European green crab Carcinus maenas invasion. Marine Ecology Progress Series, 548: 31–45.
Mathieson AC, Moore GE, and Short FT. 2010. A floristic comparison of seaweeds from James Bay and three contiguous Northeastern Canadian Arctic sites. Rhodora, 112(952): 396–434.
McIver R, Milewski I, and Lotze HK. 2015. Land use and nitrogen loading in seven estuaries along the southern Gulf of St. Lawrence, Canada. Estuarine, Coastal and Shelf Science, 165: 137–148.
McIver R, Milewski I, Loucks R, and Smith R. 2018. Estimating nitrogen loading and far-field dispersal potential from background sources and coastal finfish aquaculture: a simple framework and case study in Atlantic Canada. Estuarine, Coastal and Shelf Science, 205: 46–57.
McIver R, Cullain N, Schmidt AL, and Lotze HK. 2019. Linking eutrophication indicators in eelgrass habitats to nitrogen loading and mitigating site characteristics in eastern New Brunswick, Canada. Marine Environmental Research, 144: 141–153.
McKenzie LJ, Nordlund LM, Jones BL, Cullen-Unsworth LC, Roelfsema C, and Unsworth RKF. 2020. The global distribution of seagrass meadows. Environmental Research Letters, 15: 074041.
McKone KL, and Tanner CE. 2009. Role of salinity in the susceptibility of eelgrass Zostera marina to the wasting disease pathogen Labyrinthula zosterae. Marine Ecology Progress Series, 377: 123–130.
McRoy PC. 1969. Eelgrass under arctic winter ice. Nature, 224: 818–819.
Mersey Tobeatic Research Institute (MTRI) and Parks Canada. 2016. Annual Report of Research and Monitoring in the Greater Kejimkujik Ecosystem 2016. Mersey Tobeatic Research Institute (MTRI), Kempt, Nova Scotia. 124 p.
Micheli F, and Halpern BS. 2005. Low functional redundancy in coastal marine assemblages. Ecology Letters, 8: 391–400.
Mills KE, Pershing AJ, Brown CJ, Chen Y, Chiang F-S, Holland DS, et al. 2013. Fisheries management in a changing climate: lessons from the 2012 ocean heat wave in the Northwest Atlantic. Oceanography, 26(2): 191–195.
Minister of Fisheries, Oceans and the Canadian Coast Guard Mandate Letter. 2019 [online]: Available from pm.gc.ca/en/mandate-letters/2019/12/13/minister-fisheries-oceans-and-canadian-coast-guard-mandate-letter.
Minns CK, Randall RG, Smokorowski KE, Clarke KD, Velez-Espino A, Gregory RS, et al. 2011. Direct and indirect estimates of the productive capacity of fish habitat under Canada’s Policy for the Management of Fish Habitat: where have we been, where are we now, and where are we going? Canadian Journal of Fisheries and Aquatic Sciences, 68: 2204–2227.
Moore KA, Neckles HA, and Orth RJ. 1996. Zostera marina (eelgrass) growth and survival along a gradient of nutrients and turbidity in the lower Chesapeake Bay. Marine Ecology Progress Series, 142: 247–259.
Morris CJ, Gregory RS, Laurel BJ, Methven DA, and Warren MA. 2011. Potential effect of eelgrass (Zostera marina) loss on nearshore Newfoundland fish communities, due to invasive green crab (Carcinus maenas). Canadian Scientific Advice Secretariat Research Document 2010/140.
Muehlstein LK. 1989. Perspectives on the wasting disease of eelgrass Zostera marina. Diseases of Aquatic Organisms, 7(3): 211–221.
Muehlstein LK. 1992. The host-pathogen interaction in the wasting disease of eelgrass, Zostera marina. Canadian Journal of Botany, 70(10): 2081–2088.
Muehlstein LK, Porter D, and Short FT. 1991. Labyrinthula zosterae sp. nov., the causative agent of wasting disease of eelgrass, Zostera marina. Mycologia, 83(2): 180–191.
Murphy GEP, Wong MC, and Lotze HK. 2019. A human impact metric for coastal ecosystems with application to seagrass beds in Atlantic Canada. FACETS, 4(1): 210–237.
Nagel EJ, Murphy G, Wong MC, and Lotze HK. 2018. Nitrogen loading rates for twenty-one seagrass inhabited bays in Nova Scotia, Canada. Canadian Technical Report of Fisheries and Aquatic Sciences 3260. Fisheries and Oceans Canada.
Namba M, Lotze HK, and Schmidt AL. 2018. Large-scale differences in community structure and ecosystem services of eelgrass (Zostera marina) beds across three regions in Eastern Canada. Estuaries and Coasts, 41(1): 177–192.
NASA/GSFC OBPG. 2019. Chlorophyll-a, Aqua MODIS, NPP, L3SMI, Global, 4km, Science Quality, 2003-present (Monthly Composite). NOAA, NMFS, SWFSC, and ERD [online]: Available from coastwatch.pfeg.noaa.gov/erddap/griddap/erdMH1chlamday.html.
Nejrup LB, and Pedersen MF. 2008. Effects of salinity and water temperature on the ecological performance of Zostera marina. Aquatic Botany, 88(3): 239–246.
NOAA ERD and CoastWatch West Coast Regional Node. 2019. Multi-scale Ultra-high Resolution (MUR) SST Analysis fv04.1, Global, 0.01°, 2002-present, Monthly [online]: Available from coastwatch.pfeg.noaa.gov/erddap/griddap/jplMURSST41mday.html.
Norling K, Rosenberg R, Hulth S, Grémare A, and Bonsdorff E. 2007. Importance of functional biodiversity and species-specific traits of benthic fauna for ecosystem functions in marine sediments. Marine Ecology Progress Series, 332: 11–23.
Olesen B. 1999. Reproduction in Danish eelgrass (Zostera marina L.) stands: size-dependence and biomass partitioning. Aquatic Botany, 65(1–4): 209–219.
Olesen B, and Sand-Jensen K. 1993. Seasonal acclimatization of eelgrass Zostera marina growth to light. Marine Ecology Progress Series, 94: 91–99.
Olesen B, and Sand-Jensen K. 1994. Patch dynamics of eelgrass Zostera marina. Marine Ecology Progress Series, 106: 147–156.
Olesen B, Krause-Jensen D, Marbà N, and Christensen PB. 2015. Eelgrass Zostera marina in subarctic Greenland: dense meadows with slow biomass turnover in cold waters. Marine Ecology Progress Series, 518: 107–121.
Olsen JL, Rouzé P, Verhelst B, Lin Y-C, Bayer T, Collen J, et al. 2016. The genome of the seagrass Zostera marina reveals angiosperm adaptation to the sea. Nature, 530(7590): 331–335.
Olson A. 2017. Seagrass meadows as seascape nurseries for rockfish (Sebastes spp.). M.Sc. thesis, University of Victoria.
Olson AM, Hessing‐Lewis M, Haggarty D, and Juanes F. 2019. Nearshore seascape connectivity enhances seagrass meadow nursery function. Ecological Applications, 29(5): e01897.
O’Neill JD, and Costa M. 2013. Mapping eelgrass (Zostera marina) in the Gulf Islands National Park Reserve of Canada using high spatial resolution satellite and airborne imagery. Remote Sensing of Environment, 133: 152–167.
Orth RJ, Carruthers TJB, Dennison WC, Duarte CM, Fourqurean JW, Heck KL, et al. 2006. A global crisis for seagrass ecosystems. BioScience, 56(12): 987–996.
Pan J, Jiang X, Li X, Cong Y, Zhang Z, Li Z, et al. 2011. Influence of temperature and salinity on germination of eelgrass (Zostera marina L.) seeds. Journal of Ocean University of China, 10: 147–152.
Pease BC. 1974. Effects of log dumping and rafting on the marine environment of southeast Alaska. PNW-GTR-022. US Department of Agriculture, Forest Service, Pacific Northwest Forest and Range Experiment Station, Portland, Oregon.
Pedersen O, Binzer T, and Borum J. 2004. Sulphide intrusion in eelgrass (Zostera marina L.). Plant, Cell & Environment, 27(5): 595–602.
Penhale PA, and Wetzel RG. 1983. Structural and functional adaptations of eelgrass (Zostera marina L.) to the anaerobic sediment environment. Canadian Journal of Botany, 61: 1421–1428.
Petchey OL, and Gaston KJ. 2006. Functional diversity: back to basics and looking forward. Ecology Letters, 9: 741–758.
Phillips RC, Grant WS, and McRoy PC. 1983a. Reproductive strategies of eelgrass (Zostera marina L.). Aquatic Botany, 16: 1–20.
Phillips RC, McMillian C, and Bridges KW. 1983b. Phenology of eelgrass, Zostera marina L. along latitudinal gradients in North America. Aquatic Botany, 15: 145–156.
Postlethwaite VR, McGowan AE, Kohfeld KE, Robinson CLK, and Pellatt MG. 2018. Low blue carbon storage in eelgrass (Zostera marina) meadows on the Pacific Coast of Canada. PLoS ONE, 13(6): e0198348.
Pulido C, and Borum J. 2010. Eelgrass (Zostera marina) tolerance to anoxia. Journal of Experimental Marine Biology and Ecology, 385: 8–13.
Quiros TEA, Croll D, Tershy C, Fortes MD, and Raimondi P. 2017. Land use is a better predictor of tropical seagrass condition than marine protection. Biological Conservation, 209: 454–463.
Ralph PJ, and Short FT. 2002. Impact of the wasting disease pathogen, Labyrinthula zosterae, on the photobiology of eelgrass Zostera marina. Marine Ecology Progress Series, 226: 265–271.
Ralph PJ, Durako MJ, Enríque S, Collier CJ, and Doblin MA. 2007. Impact of light limitation on seagrasses. Journal of Experimental Marine Biology and Ecology, 350(1–2): 176–193.
Rao AS, Gregory RS, Murray G, Ings DW, Coughlan EJ, and Newton BH. 2014. Eelgrass (Zostera marina) locations in Newfoundland and Labrador. Canadian Technical Report of Fisheries and Aquatic Sciences 3113.
Rehr AP, Williams GD, Tolimeieri N, and Levin PS. 2014. Impacts of terrestrial and shoreline stressors on eelgrass in Puget Sound: an expert elicitation. Coastal Management, 42: 246–262.
Renkawitz MD, Gregory RS, and Schneider DC. 2011. Habitat dependant growth of three species of bottom settling fish in a coastal fjord. Journal of Experimental Marine Biology and Ecology, 409(1–2): 79–88.
Reynolds PL, Stachowicz JJ, Hovel K, Boström C, Boyer K, Cusson M, et al. 2018. Latitude, temperature, and habitat complexity predict predation pressure in eelgrass beds across the Northern Hemisphere. Ecology, 99(1): 29–35.
Roberts M, Mohamedali T, Sackmann B, Khangaonkar T, and Long W. 2014. Puget Sound and the Straits dissolved oxygen assessment: impacts of current and future human nitrogen sources and climate change through 2070. 14-03-007. Department of Ecology, State of Washington, Olympia, Washington.
Robertson AI, and Mann KH. 1984. Disturbance by ice and life-history adaptations of the seagrass Zostera marina. Marine Biology, 80(2): 131–141.
Robinson CLK, Yakimishyn J, and Dearden P. 2011. Habitat heterogeneity in eelgrass fish assemblage diversity and turnover. Aquatic Conservation: Marine and Freshwater Ecosystems, 21(7): 625–635.
Röhr ME, Holmer M, Baum JK, Björk M, Boyer K, Chin D, et al. 2018. Blue carbon storage capacity of temperate eelgrass (Zostera marina) meadows. Global Biogeochemical Cycles, 32(10): 1457–1475.
Roman J. 2006. Diluting the founder effect: cryptic invasions expand a marine invader’s range. Proceedings of the Royal Society B: Biological Sciences, 273: 2453–2459.
Ruesink JL, Stachowicz JJ, Reynolds PL, Boström C, Cusson M, Douglass J, et al. 2018. Form-function relationships in a marine foundation species depend on scale: a shoot to global perspective from a distributed ecological experiment. Oikos, 127(3): 364–374.
Ryan MR, Killen SS, Gregory RS, and Snelgrove PVR. 2012. Predators and distance between habitat patches modify gap crossing behaviour of juvenile Atlantic cod (Gadus morhua, L. 1758). Journal of Experimental Marine Biology and Ecology, 422: 81–87.
Salo T, and Pedersen MF. 2014. Synergistic effects of altered salinity and temperature on estuarine eelgrass (Zostera marina) seedlings and clonal shoots. Journal of Experimental Marine Biology and Ecology, 457: 143–150.
Salo T, Pedersen MF, and Boström C. 2014. Population specific salinity tolerance in eelgrass (Zostera marina). Journal of Experimental Marine Biology and Ecology, 461: 425–429.
Schein A, Courtenay SC, Kidd KA, Campbell A, and van den Heuval MR. 2013. Food web structure within an estuary of the southern Gulf of St. Lawrence undergoing eutrophication. Canadian Journal of Fisheries and Aquatic Sciences, 70: 1805–1812.
Schmidt AL, Coll M, Romanuk TN, and Lotze H. 2011. Ecosystem structure and services in eelgrass Zostera marina and rockweed Ascophyllum nodosum habitats. Marine Ecology Progress Series, 437: 51–68.
Schmidt AL, Wysmyk JKC, Craig SE, and Lotze HK. 2012. Regional-scale effects of eutrophication on ecosystem structure and services of seagrass beds. Limnology and Oceanography, 57(5): 1389–1402.
Schmidt AL, Coll M, and Lotze HK. 2017. Regional-scale differences in eutrophication effects on eelgrass-associated (Zostera marina) macrofauna. Estuaries and Coasts, 40(4): 1096–1112.
Schneider DC, Norris MJ, and Gregory RS. 2008. Predictive analysis of scale-dependent habitat association: juvenile cod (Gadus spp.) in eastern Newfoundland. Estuarine and Coastal Shelf Science, 79: 71–78.
Seagrass Conservation Working Group (SCWG). 2020 [online]: Available from seagrassconservation.org.
Sedell JR, Leone F, and Duval W. 1991. Water transportation and storage of logs. In Influences of forest and rangeland management on salmonid fishes and their habitats. Edited by W Meehan. Special Publication 19. American Fisheries Society, Bethesda, Maryland. pp. 325–368.
Segovia BT, Sanders RD, Morien E, Adamczyk EM, Forbes C, Hessing-Lewis M, et al. In review. Diversity patterns of epibiotic microeukaryotes associated with Zostera marina leaves in the cost of British Columbia (Canada). Environmental Microbiology.
Seymour NR, Miller AG, and Garbary DJ. 2002. Decline of Canada geese (Branta canadensis) and common goldeneye (Bucephala clangula) associated with a collapse of eelgrass (Zostera marina) in a Nova Scotia estuary. Helgoland Marine Research, 56: 198–202.
Shafer DJ, Kaldy JE, and Gaeckle JL. 2014. Science and management of the introduced seagrass Zostera japonica in North America. Environmental Management, 53: 147–162.
Sharpe C, Carr‐Harris C, Arbeider M, Wilson SM, and Moore JW. 2019. Estuary habitat associations for juvenile Pacific salmon and pelagic fish: implications for coastal planning processes. Aquatic Conservation: Marine and Freshwater Ecosystems, 29: 1636–1656.
Short FT. 1987. Effects of sediment nutrients on seagrasses: literature review and mesocosm experiment. Aquatic Botany, 27: 41–57.
Short FT, and Neckles HA. 1999. The effects of global climate change on seagrasses. Aquatic Botany, 63: 169–196.
Short FT, Mathieson AC, and Nelson JI. 1986. Recurrence of the eelgrass wasting disease at the border of New Hampshire and Maine, USA. Marine Ecology Progress Series, 29: 89–92.
Short FT, Burdick DM, and Kaldy JE. 1995. Mesocosm experiments quantify the effects of eutrophication on eelgrass, Zostera marina. Limnology and Oceanography, 40(4): 740–749.
Short FT, Polidoro B, Livingstone SR, Carpenter KE, Bandeira S, Bujang JS, et al. 2011. Extinction risk assessment of the world’s seagrass species. Biological Conservation, 144(7): 1961–1971.
Siegle MR, Robinson CL, and Yakimishyn J. 2014. The effect of region, body size, and sample size on the weight-length relationships of small-bodied fishes found in eelgrass meadows. Northwest Science, 88(2): 140–154.
Skinner MA, Courtenay SC, and McKindsey CW. 2013. Reductions in distribution, photosynthesis, and productivity of eelgrass Zostera marina associated with oyster Crassostrea virginica aquaculture. Marine Ecology Progress Series, 486: 105–119.
Spalding MD, Fox HE, Allen GR, Davidson N, Ferdana ZA, Finlayson M, et al. 2007. Marine ecoregions of the world: a bioregionalization of coastal and shelf areas. BioScience, 57: 573–583.
Staehr PA, and Borum J. 2011. Seasonal acclimation in metabolism reduces light requirements of eelgrass (Zostera marina). Journal of Experimental Marine Biology and Ecology, 407(2): 139–146.
Stark KA, Thompson PL, Yakimishyn J, Lee L, Adamczyk EM, Hessing-Lewis M, et al. 2020. Beyond a single patch: local and regional processes explain diversity patterns in a seagrass epifaunal metacommunity. Marine Ecology Progress Series.
Stewart DB, and Lockhart WL. 2004. Summary of the Hudson Bay marine ecosystem overview. Prepared by Arctic Biological Consultants, Winnipeg, Manitoba, for Canada Department of Fisheries and Oceans, Winnipeg, Manitoba.
Stuart-Smith RD, Bates AE, Lefcheck JS, Duffy JE, Baker SC, Thomson RJ, et al. 2013. Integrating abundance and functional traits reveals new global hotspots of fish diversity. Nature, 501: 539–542.
Sullivan BK, Sherman TD, Damare VS, Lilje O, and Gleason FH. 2013. Potential roles of Labyrinthula spp. in global seagrass population declines. Fungal Ecology, 6(5): 328–338.
Sutton JN, Johannessen SC, and Macdonald RW. 2013. A nitrogen budget for the Strait of Georgia, British Columbia, with emphasis on particulate nitrogen and dissolved inorganic nitrogen. Biogeosciences, 10: 7179–7194.
Talbot SL, Sage GK, Rearick JR, Fowler MC, Muñiz-Salazar R, Baibak B, et al. 2016. The structure of genetic diversity in eelgrass (Zostera marina L.) along the North Pacific and Bering Sea Coasts of Alaska. PLoS ONE, 11(4): e0152701.
Thistle ME, Schneider DC, Gregory RS, and Wells NJ. 2010. Fractal measures of habitat fragmentation: maximum densities of juvenile cod occur at intermediate eelgrass complexity. Marine Ecology Progress Series, 405: 39–56.
Thom RM, Borde AB, Rumrill S, Woodruff DL, Williams GD, Southard JA, et al. 2003. Factors influencing spatial and annual variability in eelgrass (Zostera marina L.) meadows in Willapa Bay, Washington, and Coos Bay, Oregon, estuaries. Estuaries, 26: 1117–1129.
Touchette BW, Burkholder JM, and Glasgow HB. 2003. Variations in eelgrass (Zostera marina L.) morphology and internal nutrient composition as influenced by increased temperature and water column nitrate. Estuaries, 26(1): 142–155.
Tupper M, and Boutilier RG. 1995. Effects of habitat on settlement, growth, and postsettlement survival of Atlantic cod (Gadus morhua). Canadian Journal of Fisheries and Aquatic Sciences, 52(9): 1834–1841.
Unsworth RKF, McKenzie LJ, Collier CJ, Cullen-Unsworth LC, Duarte CM, Eklöf JS, et al. 2019. Global challenges for seagrass conservation. Ambio, 48(8): 801–815.
van den Heuvel MR, Hitchcock JK, Coffin MRS, Pater CC, and Courtenary SC. 2019. Inorganic nitrogen has a dominant impact on estuarine eelgrass distribution in the Southern Gulf of St. Lawrence, Canada. Limnology and Oceanography, 64(6): 2313–2327.
van der Heide T, Peeters ETHM, Hermus DCR, van Katwijk MM, Roelofs JGM, and Smolders AJP. 2009. Predicting habitat suitability in temperate seagrass ecosystems. Limnology and Oceanography, 54(6): 2018–2024.
van Katwijk MM, Vergeer LHT, Schmitz GHW, and Roelofs JGM. 1997. Ammonium toxicity in eelgrass Zostera marina. Marine Ecology Progress Series, 157: 159–173.
van Katwijk MM, van der Welle MEW, Lucassen ECHET, Vonk JA, Christianen MJA, Kiswara W, et al. 2011. Early warning indicators for river nutrient and sediment loads in tropical seagrass beds: a benchmark from a near-pristine archipelago in Indonesia. Marine Pollution Bulletin, 62: 1512–1520.
Vandermeulen H. 2014. Bay-scale assessment of eelgrass beds using sidescan and video. Helgoland Marine Research, 68(4): 559–569.
Waldbusser GG, and Salisbury JE. 2014. Ocean acidification in the coastal zone from an organism’s perspective: multiple system parameters, frequency domains, and habitats. Annual Review of Marine Science, 6: 221–247.
Warren MA, Gregory RS, Laurel BJ, and Snelgrove PVR. 2010. Increasing density of juvenile Atlantic (Gadus morhua) and Greenland cod (G. ogac) in association with spatial expansion and recovery of eelgrass (Zostera marina) in a coastal nursery habitat. Journal of Experimental Marine Biology and Ecology, 394(1–2): 154–160.
Waycott M, Duarte CM, Carruthers TJB, Orth RJ, Dennison WC, Olyarnik S, et al. 2009. Accelerating loss of seagrasses across the globe threatens coastal ecosystems. Proceedings of the National Academy of Sciences of the United States of America, 106(30): 12377–12381.
Webster T, McGuigan K, Crowell N, Collins K, and MacDonald C. 2016. Optimization of data collection and refinement of post-processing techniques for Maritime Canada’s first shallow water topographic-bathymetric lidar survey. Journal of Coastal Research, 76: 31–43.
Weldon J, Courtenay S, and Garbary D. 2009. The community aquatic monitoring program (CAMP) for measuring marine environmental health in coastal waters of the southern Gulf of St. Lawrence: 2007 overview. Canadian Technical Report of Fisheries and Aquatic Sciences 2825. viii + 75 p.
Whippo R, Knight NS, Prentice C, Cristiani J, Siegle MR, and O’Connor MI. 2018. Epifaunal diversity patterns within and among seagrass meadows suggest landscape-scale biodiversity processes. Ecosphere, 9(11): e02490.
Williams SL. 2007. Introduced species in seagrass ecosystems: status and concerns. Journal of Experimental Marine Biology and Ecology, 350: 89–110.
Wilson KL, and Lotze HK. 2019. Climate change projections reveal range shifts of eelgrass Zostera marina in the Northwest Atlantic. Marine Ecology Progress Series, 620: 47–62.
Wilson KL, Skinner MA, and Lotze HK. 2019. Eelgrass (Zostera marina) and benthic habitat mapping in Atlantic Canada using high-resolution SPOT 6/7 satellite imagery. Estuarine, Coastal and Shelf Science, 226: 106292.
Wong MC. 2018. Secondary production of macrobenthic communities in seagrass (Zostera marina, eelgrass) beds and bare soft sediments across differing environmental conditions in Atlantic Canada. Estuaries and Coasts, 41(2): 536–548.
Wong MC, and Dowd M. 2015. Patterns in taxonomic and functional diversity of microbenthic invertebrates across seagrass habitats: a case study in Atlantic Canada. Estuaries and Coasts, 38: 2323–2336.
Wong MC, and Dowd M. 2016. A model framework to determine the production potential of fish derived from coastal habitats for use in habitat restoration. Estuaries and Coasts, 39: 1785–1800.
Wong MC, and Kay LM. 2019. Partial congruence in habitat patterns for taxonomic and functional diversity of fish assemblages in seagrass ecosystems. Marine Biology, 166(4): 46.
Wong MC, and Vercaemer B. 2012. Effects of invasive colonial tunicates and a native sponge on the growth, survival, and light attenuation of eelgrass (Zostera marina). Aquatic Invasions, 7: 315–326.
Wong MC, Bravo MA, and Dowd M. 2013. Ecological dynamics of Zostera marina (eelgrass) in three adjacent bays in Atlantic Canada. Botanica Marina, 56: 413–424.
Wong MC, Griffiths G, and Vercaemer B. 2020. Seasonal response and recovery of eelgrass (Zostera marina) to short-term reductions in light availability. Estuaries and Coasts, 43: 120–134.
Wu PP, Mengersen K, McMahon K, Kendrick GA, Chartrand K, York PH, et al. 2017. Timing anthropogenic stressors to mitigate their impact on marine ecosystem resilience. Nature Communications, 8: 1263.
Xu S, Zhou Y, Wang P, Wang F, Zhang X, and Gu R. 2016. Salinity and temperature significantly influence seed germination, seedling establishment, and seedling growth of eelgrass Zostera marina L. PeerJ, 4: e2697.
Yang S, Wheat EE, Horwith MJ, and Ruesink JL. 2013. Relative impacts of natural stressors on life history traits underlying resilience of intertidal eelgrass (Zostera marina L.). Estuaries and Coasts, 36(5): 1006–1013.
Zimmerman R, Hill V, Jinuntuya M, Celebi B, Ruble D, Smith M, et al. 2017. Experimental impacts of climate warming and ocean carbonation on eelgrass Zostera marina. Marine Ecology Progress Series, 566: 1–15.
Appendix A—Supplementary species assemblage survey information
Differences in study objectives and in sampling methods make comparison of species assemblages in eelgrass meadows across Canada a challenge. Along the Scotian Shelf and Gulf of St. Lawrence, eelgrass surveys have focused on infauna, benthic invertebrate, and fish communities (Joseph et al. 2006; Schmidt et al. 2011, 2017; Coffin et al. 2018; Cullain et al. 2018b; Wong 2018; Wong and Kay 2019), whereas surveys in Newfoundland have primarily focused on fish assemblages (Warren et al. 2010; Cote et al. 2013; Laurel et al. 2017). On the Pacific coast, eelgrass surveys have focused on epifaunal invertebrate (Duffy et al. 2015; Whippo et al. 2018; Stark et al. 2020) and fish assemblages (Robinson et al. 2011; Iacarella et al. 2018; Olson et al. 2019), with fewer standardized surveys of infaunal communities (Harrison 1987; Berkenbusch et al. 2007). Although fish assemblages have been surveyed across bioregions, there are notable differences in sampling techniques. For example, most fish surveys along the Scotian Shelf have used underwater visual census or benthic trawls (Schmidt et al. 2017; Wong and Kay 2019), while beach seining has been commonly employed in the Gulf of St. Lawrence, Newfoundland (Laurel et al. 2003a, 2017; Joseph et al. 2006; Weldon et al. 2009; Cote et al. 2013), and Pacific coast (Robinson et al. 2011; Iacarella et al. 2018; Reynolds et al. 2018). Yet, significantly different fish species assemblages have been reported when comparing beach seines versus underwater visual census or baited-camera surveys (Baker et al. 2016; Dalley et al. 2017), because different techniques under- or overestimate certain species.
Supplementary material
Supplementary Material 1 (DOCX / 873 KB)
- Download
- 873.17 KB
Information & Authors
Information
Published In
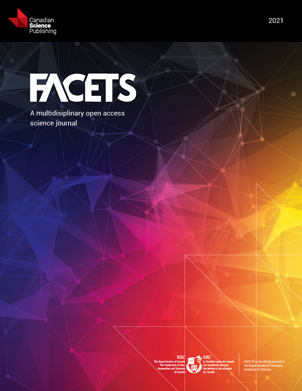
FACETS
Volume 6 • Number 1 • January 2021
Pages: 139 - 179
Editor: Daniel E. Schindler
History
Received: 11 March 2020
Accepted: 15 October 2020
Version of record online: 11 February 2021
Copyright
© 2021 Her Majesty the Queen in Right of Canada, Jillian Dunic, Emily Adamczyk, Sarah Bittick, Isabelle Côté, John Cristiani, Emilie Geissinger, Heike Lotze, Mary O’Connor, and Carlos Araújo. This work is licensed under a Creative Commons Attribution 4.0 International License (CC BY 4.0), which permits unrestricted use, distribution, and reproduction in any medium, provided the original author(s) and source are credited.
Data Availability Statement
All relevant data are within the paper and in the Supplementary Material.
Key Words
Sections
Subjects
Authors
Author Contributions
All conceived and designed the study.
All contributed resources.
All drafted or revised the manuscript.
Competing Interests
The authors have declared that no competing interests exist.
Metrics & Citations
Metrics
Other Metrics
Citations
Cite As
Grace E.P. Murphy, Jillian C. Dunic, Emily M. Adamczyk, Sarah J. Bittick, Isabelle M. Côté, John Cristiani, Emilie A. Geissinger, Robert S. Gregory, Heike K. Lotze, Mary I. O’Connor, Carlos A.S. Araújo, Emily M. Rubidge, Nadine D. Templeman, and Melisa C. Wong. 2021. From coast to coast to coast: ecology and management of seagrass ecosystems across Canada. FACETS.
6: 139-179.
https://doi.org/10.1139/facets-2020-0020
Export Citations
If you have the appropriate software installed, you can download article citation data to the citation manager of your choice. Simply select your manager software from the list below and click Download.
Cited by
1. Changes in sediment regimes and mass accumulation rates in Eeyou Istchee eelgrass habitat, James Bay, Canada
2. Influence of Nitrogen Bioavailability on the Anaerobic Co-Digestion of the Aegagropiles of the Seagrass Posidonia oceanica with Different Nitrogen-Rich Substrates: Process Performance and Kinetic Analysis
3. Comparing demersal fish and large mobile decapod crustacean assemblages in nearshore marine habitats across a boreal-sub-Arctic gradient using baited cameras
4. Role of seagrass physical structure in macrofaunal biodiversity-ecosystem functioning relationships
5. Anthropogenic impacts on seagrass habitat connectivity: a model to explore potential links between human activity and marine invertebrate metapopulation persistence
6. Incidence of herbivory of the invasive crab Percnon gibbesi on the endemic Mediterranean seagrass Posidonia oceanica
7. Eelgrass (Zostera marina) Trait Variation Across Varying Temperature-Light Regimes
8. Functional Redundancy of Primary Producers Mitigates Function Loss in an Ice-Scoured Eelgrass Meadow (Zostera Marina, Linnaeus 1758)
9. Microplastic pollution on seagrass blades in two coastal bays in Northern Mindanao, Philippines
10. From oceans apart to the global ocean: Including marine connectivity in global conservation targets
11. From land to deep sea: A continuum of cumulative human impacts on marine habitats in Atlantic Canada
12. Incidence of herbivory of the invasive crab Percnon gibbesi on the endemic Mediterranean seagrass Posidonia oceanica
13. Thirty years of change: Assessing the dynamics of fish communities in Daya Bay, a semi-enclosed coastal ecosystem of the South China sea
14. Effects of light and water column nutrient availability on eelgrass Zostera marina productivity in Eeyou Istchee, eastern James Bay, Quebec
15. The “Turning Point” for the Fall Goose Hunt in Eeyou Istchee: A Social-Ecological Regime Shift from an Indigenous Knowledge Perspective
16. Variation in genomic vulnerability to climate change across temperate populations of eelgrass (
Zostera marina
)
17. Mapping and Characterizing Eelgrass Meadows Using UAV Imagery in Placentia Bay and Trinity Bay, Newfoundland and Labrador, Canada
18. Predicted shifts in suitable habitat of interacting benthic species in a warmer and invaded Canadian Arctic
19. Simulating dispersal in a complex coastal environment: the Eastern Shore Islands archipelago
20. River influence on mercury bioaccumulation in the coastal food web of Eeyou Istchee, James Bay, Canada
21. Decades of eelgrass meadow dynamics across the northeast Pacific support seascape-scale conservation
22. The Role of Boreal Seagrass Meadows in the Coastal Filter
23. Hunting and seagrass affect fall stopover Canada goose distribution in eastern Canada
24. Ocean current patterns drive the worldwide colonization of eelgrass (Zostera marina)
25. Effects of disturbance on macrofaunal
biodiversity‐ecosystem
functioning relationships in seagrass habitats
26. Coastal community perceptions of eelgrass in Atlantic Canada: Considerations for management
27. Application of Deep Learning for Classification of Intertidal Eelgrass from Drone-Acquired Imagery
28. Estimation of Seagrass Beds Changes in the Barugaiya Village Waters over a Period of 5 Years 2019-2023
29. Predator density, not structure, influences intraspecific competition in the mud crab Dyspanopeus sayi
30. Posidonia oceanica restoration review: Factors affecting seedlings
31. The reproducibility of remotely piloted aircraft systems to monitor seasonal variation in submerged seagrass and estuarine habitats
32. Public perceptions of cultural ecosystem services provided by beach nourishment and eelgrass restoration in southern Sweden
33. Cumulative effects of long-term anthropogenic impacts on resident fish communities in subarctic fishing harbours
34. Fine-scale ensemble species distribution modeling of eelgrass (Zostera marina) to inform nearshore conservation planning and habitat management
35. The evolutionary past and the uncertain future of foundational species
36. Seasonally and Spatially Variable Organic Matter Contributions From Watershed, Marine Macrophyte, and Pelagic Sources to the Northeast Pacific Coastal Ocean Margin
37. Empirical Remote Sensing Algorithms to Retrieve SPM and CDOM in Québec Coastal Waters
38. Aquaculture and eelgrass Zostera marina interactions in temperate ecosystems
39. Photoacclimation and Light Thresholds for Cold Temperate Seagrasses
40. A climate-resilient marine conservation network for Canada
41. Incorporating anthropogenic thresholds to improve understanding of cumulative effects on seagrass beds
42. Variability of bio-optical properties in nearshore waters of the estuary and Gulf of St. Lawrence: Absorption and backscattering coefficients
43. Decadal increase in the ecological status of a North-Atlantic intertidal seagrass meadow observed with multi-mission satellite time-series
44. A Biophysical Model and Network Analysis of Invertebrate Community Dispersal Reveals Regional Patterns of Seagrass Habitat Connectivity
45. Using Landsat Time-Series to Monitor and Inform Seagrass Dynamics: A Case Study in the Tabusintac Estuary, New Brunswick, Canada