The effect of global climate change on the future distribution of economically important macroalgae (seaweeds) in the northwest Atlantic
Abstract
An increase in greenhouse gas emissions has led to a rise in average global air and ocean temperatures. Increased sea surface temperatures can cause changes in species’ distributions, particularly those species close to their thermal tolerance limits. We use a bioclimate envelope approach to assess potential shifts in the range of marine macroalgae harvested in North American waters: rockweed (Fucus vesiculosus Linnaeus, 1753), serrated wrack (Fucus serratus Linnaeus, 1753), knotted wrack (Ascophyllum nodosum (Linnaeus) Le Jolis, 1863), carrageen moss (Chondrus crispus Stackhouse, 1797), and three kelp species (Laminaria digitata (Hudson) J.V. Lamouroux, 1813; Saccharina latissima (Linnaeus) C.E. Lane, C. Mayes, Druehl et G.W. Saunders, 2006; and Saccharina longicruris (Bachelot de la Pylaie) Kuntze, 1891). We determined species’ thermal limits from the current sea surface temperatures associated with their geographical distributions. Future distributions were based on sea surface temperatures projected for the year ∼2100 by four atmosphere-ocean general circulation models and earth system models for regional concentration pathways (RCPs) 4.5 and 8.5. Future distributions based on RCP 8.5 indicate that the presence of all but rockweed (F. vesiculosus) is likely to be threatened by warming waters in the Gulf of St. Lawrence and along the Atlantic coast of Nova Scotia. Range retractions of macroalgae will have significant ecological and economic effects including impacts on commercial fisheries and harvest rates and losses of floral and faunal biodiversity and production, and should be considered in the designation of marine protected areas.
Introduction
With unprecedented increases in greenhouse gas emissions and a warming climate, marine ecosystems will incur major changes in ecological structure and function. Species are likely to react in one of three ways: adapting, migrating to more appropriate climate conditions, or becoming extirpated from their original distribution (Walther et al. 2002; Parmesan and Yohe 2003; Parmesan 2006). Climate change has already had observable impacts on the distribution of marine species including seaweeds (Perry et al. 2010). Seaweeds (hereafter referred to as macroalgae) are particularly sensitive as the adult form of many species is sessile. Indeed, a poleward range retraction of kelp has been observed on the coasts of Spain (Fernández 2011; Díez et al. 2012), Portugal (Tuya et al. 2012), France (Cosson 1999), Norway (Moy and Christie 2012), Australia (Wernberg et al. 2011), and Japan (Tanaka et al. 2012). Expansion of macroalgal ranges is dependent on the dispersal of spores to appropriate habitats and there is evidence that this is occurring. Poleward range extensions of rockweed (Fucus vesiculosus Linnaeus, 1753) (Jueterbock et al. 2013; Nicastro et al. 2013), a brown seaweed species (Bifurcaria bifurcata R. Ross, 1958), a bushy seaweed species (Cystoseira tamariscifolia (Hudson) Papenfuss, 1950) (Mieszkowska et al. 2006), and a kelp species Laminaria hyperborea (Gunnerus) Foslie, 1884 (Müller et al. 2009) have been linked to increasing temperatures. Although some macroalgae can adapt to warming through northward shifts of their range, it can be at the expense of overall distribution and loss of genetic diversity within a species (Nicastro et al. 2013).
Local extirpation of macroalgae, or even reduced production due to warming stress, will have broad ecological impacts. Macroalgae include foundation species that provide habitat for other taxa. The canopy and understory of macroalgae provide shelter and nursery grounds for a large number of species, including those with direct economic importance (e.g., Rangeley and Kramer 1995; Steneck et al. 2002; Bartsch et al. 2008; Christie et al. 2009). Macroalgal communities are highly productive ecosystems fueling high rates of secondary production (Mann 1973; Fredriksen 2003; Krumhansl and Scheibling 2012).
Macroalgae have considerable direct economic importance. Globally, they provide goods and services for human consumption, fertilizers, animal feed additives, pet foods, and cosmetics (Rebours et al. 2014; Ugarte and Sharp 2012). On the eastern coast of Canada artisanal and commercial harvesting occurs in all five of the Atlantic provinces (Chopin and Ugarte 2006).
In this study we consider the fate of these economically important species with climate change in the northwest Atlantic as demonstrated in a companion study by Khan et al. (2013, fig. 1). Because temperature has been linked to changes in macroalgal distributions elsewhere, we apply a bioclimate envelope approach based on current sea surface temperatures (SSTs) and temperatures projected from global climate models to project future distributions. We consider the following species: serrated wrack (Fucus serratus Linnaeus, 1753), rockweed (F. vesiculosus), knotted wrack (Ascophyllum nodosum (Linnaeus) Le Jolis, 1863), carrageen moss (Chondrus crispus Stackhouse, 1797), and three kelps, Laminaria digitata (Hudson) J.V. Lamouroux, 1813; Saccharina latissima (Linnaeus) C.E. Lane, C. Mayes, Druehl et G.W. Saunders, 2006; and Saccharina longicruris (Bachelot de la Pylaie) Kuntze, 1891. Because air temperatures are highly correlated with sea surface temperatures (Cayan 1980; Galbraith and Larouche 2013) our bioclimate envelope should also be a proxy for intertidal macroalgae sensitive to extremes in air temperature (e.g., Schonbeck and Norton 1978).
Methods
Although a bioclimate envelope does not include all factors responsible for a species’ distribution, it provides a useful conservation tool at our sub-continental scale of investigation (Watling et al. 2013; Torossian et al. 2016). We developed bioclimate envelopes using the SSTs associated with the bathymetric and latitudinal limits of each species in the northwest Atlantic. We used daily SST data for the period 1982–1999 available in the Group for High Resolution Sea Surface Temperature (GHRSST) database (Reynolds et al. 2007). The GHRSST data are produced on a 0.25° grid and represents the surface 10 m. The data were downloaded from the Physical Oceanography Distributed Active Archive Center (podaac.jpl.nasa.gov/dataset/NCDC-L4LRblend-GLOB-AVHRR_OI), imported as network common data form (NetCDF) files, converted to raster, and clipped to include the boundaries of the study area. We selected the months of February and August, which generally represent extremes in winter and summer SSTs within the target region. Hudson Bay was excluded from the analysis as sea ice in this region produced anomalous SSTs. Bathymetry data were obtained from the General Bathymetric Chart of the Oceans 1-min grid, created in 2003 and updated in 2008 (gebco.net/). The current limits for the northern and southern latitudinal and bathymetric range were assessed from both reports in the literature (e.g., Taylor 1957; Sears 2002; Van Guelpen et al. 2007) and specimen holdings of the Atlantic Reference Centre of Fisheries and Oceans Canada. Present day SSTs were derived from GHRSST data as described by Khan et al. (2013). The bioclimate envelope was defined by determining the minimum February and maximum August SSTs within each species’ range (Table 1).
Table 1.
Latitudinal range (°N) | Thermal limits (°C) | |||||
---|---|---|---|---|---|---|
Species | Common name | South | North | Bathymetric limits (m) | February | August |
Saccharina longicruris | kelp | 41.3 | 79.8 | 2–60 | −1.8 | 21.5 |
Saccharina latissima | kelp | 41.3 | 79.8 | 2.5–30 | −1.8 | 21.5 |
Laminaria digitata | kelp | 41.3 | 62 | 0–40 | −1.7 | 21.5 |
Fucus vesiculosus | rockweed | 35 | 80 | 0–10 | −1.8 | 27.4 |
Chondrus crispus | Irish moss/carrageen moss | 40 | 60 | 0–20 | −1.8 | 21.8 |
Ascophyllum nodosum | knotted wrack | 41.5 | 66 | 0–10 | −1.8 | 20.1 |
Fucus serratus | serrated wrack | 44.3 | 47 | 0–10 | −1.2 | 18.1 |
Future distributions for the year 2100 were determined using SSTs projected by four different atmosphere-ocean general circulation models (AOGCMs) and earth system models (ESMs) used for the Intergovernmental Panel on Climate Change’s (IPCC) 5th Assessment Report (AR5) and the output of runs from two representative concentration pathways (RCPs), RCP 4.5 and RCP 8.5 (Khan et al. 2013, fig. 1). Model selections were based on models’ spatial resolution, ability to simulate present climate, and period of the model run including historical availability of historical projections. We used an ensemble of four AOGCMs and ESMs: the Canadian Centre for Climate Modelling and Analysis (CCCMA) CanESM2, the Met Office Hadley Centre HadGEM2-ES, the National Aeronautics and Space Administration (NASA) Goddard Institute for Space Studies (GISS) GISS-E2-R, and the Australian Commonwealth Scientific and Industrial Research Organization (CSIRO) CSIRO-Mk3.6. From each model’s output we selected two RCPs (4.5 and 8.5) used in the IPCC’s AR5. RCP 8.5 represents the most severe climate change projected, reaches a radiative forcing >8.5 W·m−2 by 2100, and continues to increase. In RCP 4.5 radiative forcing is stabilized at 4.5 W·m−2 after 2100, thus projecting less dire climate change. As model projections vary, we averaged the SSTs for each RCP projected by each of the four models to create an ensemble average (i.e., average of the data used for each map) change in SST for the target region.
Climate model SST data were obtained from the Program for Climate Model Diagnosis and Intercomparison (PCMDI) Earth System Gateway (ESG) (cmip.llnl.gov/cmip5/). Climate projections from the PCMDI ESG were downloaded as NetCDF files and were viewed through the NASA GISS Panoply program to obtain time-step information. Data were imported into a geographic information system (GIS) application as a feature layer. The files were then exported in Microsoft Excel and tabulated to calculate the average for each period for every climate model projection and pathway. We used data for February and August from the periods 1961–1999 and 2071–2100 to create change fields representing SST changes occurring at year 2100 (Fig. 1).
Fig. 1.

Spatial resolution varies among the AOGCMs and ESMs, but all change fields were scaled to 4 km ×4 km using an inverse distance-weighted function to enable comparisons to modern climatologies derived from GHRSST data. The power of the function was set to two. A minimum of eight neighbouring data points were used for each interpolation. Interpolation through land was eliminated by using a barrier mask. The barrier limited the search for neighboring data to only those input sample points on the same side of the barrier as the processed cell. Interpolation was performed by the Spatial Analyst extension in ArcMAP (ESRI V 10).
We used a GIS analysis to identify areas where both the future minimum February and maximum August SSTs are within the thermal boundaries and bathymetric limits of the species and to determine the future range of each species. The coarse resolution of the bathymetric layer made species’ ranges appear discontinuous. To compensate, the ARCGIS buffer tool was used to link a one-pixel coastal zone (∼15 km) with species depths. The current and potential future distributions were then mapped to determine potential gain or loss in each species’ range.
For each species the latitudinal expansion and contraction (in km) was assessed by determining the distance between the most northern (and southern) projected and present extents of the range. The area considered was limited to the northernmost extent of the Newfoundland–Labrador Shelf large marine ecosystem, as sea ice presents challenges for climate modelling and there is considerable uncertainty in projections of future SSTs where sea ice is prevalent.
Results
Maps of all species’ predicted distributions are shown in Figs. 2 and 3. Output from the NASA model projects less temperature increase in our target area than the Hadley, CCMA, and CSIRO models, thus, using NASA projections alone we would assume less change in species’ ranges. However, under both the RCP 8.5 and the more limited change of RCP 4.5 model projections, some retraction of range is indicated for each species. As expected, the northward retraction is greater with the greater warming projected under scenario RCP 8.5 (Fig. 4).
Fig. 2.

Fig. 3.

Fig. 4.

The present range of S. latissima, S. longicruris, L. digitata, A. nodosum, and F. vesiculosus already extends poleward beyond our study area (Taylor 1957, table 1; Sears 2002). However, three species (S. longicruris, S. latissima, and F. vesiculosus) are found at ∼80°N and there is little coastline left for poleward range expansion in the northwest Atlantic. The remaining three species are likely to have appropriate physical habitat available for poleward range expansion in the northwest Atlantic. Unfortunately, the geographical limitations of our analyses do not allow us to assess possibilities of range expansion in the northernmost regions, limiting our assessments to loss of area of these species in the northwest Atlantic (Figs. 2 and 3). In contrast, F. serratus presently occupies little coastline of the northernmost waters and may actually attain a larger range as seas warm. Under all projections new thermal habitat may become available for C. crispus, but the poleward shift will not compensate for the retraction at the southern edge of its range (Fig. 4).
Discussion
Range retractions of these macroalgae will have significant direct effects on local economies and ecology, and ecological effects will have indirect economic effects. Cascade effects may occur as loss of the macroalgal canopies negatively impact recruitment, growth, and survival of understory organisms (e.g., Bertness et al. 1999), resulting in compounded impacts. These impacts are likely to be greatest for species that have limited potential for poleward range expansion that would counterbalance range retraction. The following discussion focusses on how higher SSTs will impact these species, the habitats they provide, and harvests.
Seeley and Schlesinger (2012) reviewed the ecological services of A. nodosum, which grows attached to rocks in the intertidal and subtidal zones. It forms extensive beds that float as a canopy at high tide. Seeley and Schlesinger (2012) noted the importance of A. nodosum to other organisms. For instance the algal fronds support epiphytes, and the beds provide habitat for more than 100 invertebrate taxa that, in turn, provide prey for vertebrates. In US and Canadian waters A. nodosum beds are used as habitat by 34 fish species including the American eel and Atlantic cod. Six of these fish species are designated with special conservation status in the US, Canada, or both. Of the more than 17 bird species that utilize A. nodosum, 10 are designated with special conservation status (Seeley and Schlesinger 2012). Thus, reduced population or local extirpation of A. nodosum will further threaten already endangered species.
On the northwest Atlantic coast, F. vesiculosus can be found growing with A. nodosum, although the former is more prevalent on exposed coasts (Schmidt et al. 2011; Larsen 2012). Research on the habitat that F. vesiculosus provides on the Baltic coast revealed that the invertebrate assemblage it held had nearly twice the biomass compared with sites with no algae (Wikström and Kautsky 2007). We have found no comparable synthesis for the northwest Atlantic, but conclude that similar losses of fauna will occur where F. vesiculosus faces range retraction in the northwest Atlantic.
Smale et al. (2013) reviewed faunal diversity supported by kelp species in the northeastern Atlantic. They noted that a single kelp plant can support approximately 40 macroinvertebrate species. In addition, kelp provides habitat for other sessile flora and fauna. Kelp forests are nursery grounds for juvenile invertebrates and fish such as Atlantic cod and pollock, as well as feeding grounds for many fish species and fish predators. By extension, we can assume that the extirpation of kelp from portions of its existing range in the northwest Atlantic will have wide-reaching impacts on marine species diversity and production (Wilson et al. 2015).
Populations in warmer water that have not been extirpated may still be affected. Where temperatures remain or approach the optimal range, production will be maintained or enhanced (as demonstrated by experiments conducted by Wilson et al. 2015). However, populations located at the new, warmer edge of the range (where higher temperatures resulted in a poleward shift) will be subject to heat stress, which results in decreased production.
Warmer waters will have indirect impacts on the macroalgae as a habitat or as a harvestable resource by enabling expansion of invasive species that act as competitors, herbivores, or disease agents, as well as other herbivores that simply are expanding their range poleward with warming. Merzouk and Johnson (2011) provided the following example of the complex ecological interactions possible as kelp beds of the northwest Atlantic are subject to warmer waters. Warmer waters may result in increased incidence of disease in sea urchins, a major herbivore of kelp. (In high enough populations, sea urchins can decimate kelp beds.) However, if warmer waters enhance growth of invasives, such as the alga Codium Stackhouse, 1797 that reduces kelp recruitment or the encrusting alga Membranipora de Blainville, 1830 that reduces photosynthetic capacity, then kelp beds would decline (Merzouk and Johnson 2011).
Warming SSTs may affect the ability to harvest macroalgae. Harvests will no longer be possible where ranges have retracted and may no longer be economically feasible where production is decreased. Commercial harvests could be relocated poleward, but the northern portions of these macroalgal ranges are along the coasts of Newfoundland and Labrador where infrastructure, transportation networks, and labour are more limited. Thus, such geographical shifts are likely unfeasible. Local communities that harvest macroalgae from beds or collect detrital material washed up on shorelines will have to find alternative resources.
Conclusions
The most important species for commercial harvests are A. nodosum and F. vesiculosus. Where heat stress lowers their production, rates of harvests will have to be reassessed to assure that ecological values will not be compromised. This will require monitoring of productivity and air and water temperatures. Our modelled projections suggest where productivity and temperature monitoring might best be focussed. The approach that would provide the greatest benefit would be to use the projections developed from the Hadley or CCMA models that indicate the greatest warming and range retraction. For instance, populations in the Gulf of St. Lawrence and along Atlantic Nova Scotia (with the exception of F. vesiculosus) are likely to be threatened. Minimally, the future distributions predicted with the model ensemble average should be used.
These future SSTs and ranges of key macroalgal foundation species should be considered in plans for designation of future marine protected areas. As Canada strives to meet its goal of 10% of marine ecosystems protected (by 2020), consideration should be given to locations near present poleward edges of ranges as these will have the greatest chance of ensuring protection of macroalgal habitat with the inevitable climate warming.
We have not examined the possibility of algae adapted to warmer waters expanding their ranges poleward or if any have direct economic value. However even if new valuable species do appear, adaptation by industrial and artisanal users will likely be required with respect to harvest and processing methods.
Acknowledgements
Funding for this research was provided through Canada’s Climate Change Impacts and Adaptation Program Natural Resources Canada Agreements A515 and A515a and NSERC Discovery Grants to LV and GLC. We are grateful to two anonymous reviewers whose comments helped improve the manuscript.
References
Bartsch I, Wiencke C, Bischof K, Buchholz CM, Buck BH, Eggert A, et al. 2008. The genus Laminaria sensu lato: recent insights and developments. European Journal of Phycology, 43(1): 1–86.
Bertness MD, Leonard GH, Levine JM, Schmidt PR, and Ingraham AO. 1999. Testing the relative contribution of positive and negative interactions in rocky intertidal communities. Ecology, 80: 2711–2726.
Cayan DR. 1980. Large-scale relationships between sea surface temperature and surface air temperature. Monthly Weather Review, 108: 1293–1301.
Chopin T, and Ugarte R. 2006. The seaweed resource of Eastern Canada. In World seaweed resources: an authoritative reference system. Edited by AT Critchley, M Ohno, and DB Largo. A multimedia, interactive DVD-ROM. ETI BioInformatics, Amsterdam, the Netherlands. 46 p. ISBN: 90 75000 80 4.
Christie H, Norderhaug KM, and Fredriksen S. 2009. Macrophytes as habitat for fauna. Marine Ecology Progress Series, 396: 221–233.
Cosson J. 1999. Sur la disparition progressive de Laminaria digitata sur les côtes du Calvados (France). Cryptogamie Algologie, 20(1): 35–42.
Díez I, Muguerza N, Santolaria A, Ganzedo U, and Gorostiaga JM. 2012. Seaweed assemblage changes in the eastern Cantabrian Sea and their potential relationship to climate change. Estuarine, Coastal and Shelf Science, 99: 108–120.
Fernández C. 2011. The retreat of large brown seaweeds on the north coast of Spain: the case of Saccorhiza polyschides. European Journal of Phycology, 46(4): 352–360.
Fredriksen S. 2003. Food web studies in a Norwegian kelp forest based on stable isotope (δ13C and δ15N) analysis. Marine Ecology Progress Series, 260: 71–81.
Galbraith PS, and Larouche P. 2013. Chapter 1: Trends and variability in air and sea surface temperatures in eastern Canada. In Aspects of climate change in the northwest Atlantic off Canada. Edited by JW Loder, G Han, PS Galbraith, J Chassé, A van der Baaren. Canadian Technical Report of Fisheries and Aquatic Sciences, Ottawa, Ontario. Vol. 3045, x + 190 p.
Jueterbock A, Tyberghein L, Verbruggen H, Coyer JA, Olsen JL, and Hoarau G. 2013. Climate change impact on seaweed meadow distribution in the North Atlantic rocky intertidal. Ecology and Evolution, 3(5): 1356–1373.
Khan AH, Levac E, and Chmura GL. 2013. Future sea surface temperatures in Large Marine Ecosystems of the Northwest Atlantic. ICES Journal of Marine Science, 70(5): 915–921.
Krumhansl KA, and Scheibling RE. 2012. Production and fate of kelp detritus. Marine Ecology Progress Series, 467: 281–302.
Larsen PF. 2012. The macroinvertebrate fauna of rockweed (Ascophyllum nodosum)—Dominated low-energy rocky shores of the northern Gulf of Maine. Journal of Coastal Research, 28: 36–42.
Mann KH. 1973. Seaweeds—their productivity and strategy for growth: the role of large marine algae in coastal productivity is far more important than has been suspected. Science, 182: 975–981.
Merzouk A, and Johnson LE. 2011. Kelp distribution in the northwest Atlantic Ocean under a changing climate. Journal of Experimental Marine Biology and Ecology, 400: 90–98.
Mieszkowska N, Kendall MA, Hawkins SJ, Leaper R, Williamson P, Hardman-Mountford NJ, et al. 2006. Changes in the range of some common rocky shore species in Britain—a response to climate change? In Marine Biodiversity. Springer, the Netherlands. pp. 241–251.
Moy FE, and Christie H. 2012. Large-scale shift from sugar kelp (Saccharina latissima) to ephemeral algae along the south and west coast of Norway. Marine Biology Research, 8(4): 309–321.
Müller R, Laepple T, Bartsch I, and Wiencke C. 2009. Impact of oceanic warming on the distribution of seaweeds in polar and cold-temperate waters. Botanica Marina, 52(6): 617–638.
Nicastro KR, Zardi GI, Teixeira S, Neiva J, Serrão EA, and Pearson GA. 2013. Shift happens: trailing edge contraction associated with recent warming trends threatens a distinct genetic lineage in the marine macroalga Fucus vesiculosus. BMC Biology, 11: 6.
Parmesan C. 2006. Ecological and evolutionary responses to recent climate change. Annual Review of Ecology, Evolutions, and Systematics, 37: 637–669.
Parmesan C, and Yohe G. 2003. A globally coherent fingerprint of climate change impacts across natural systems. Nature, 421(6918): 37–42.
Perry RI, Cury P, Brander K, Jennings S, Möllmann C, and Planque B. 2010. Sensitivity of marine systems to climate and fishing: concepts, issues and management responses. Journal of Marine Systems, 79(3): 427–435.
Rangeley RW, and Kramer DL. 1995. Use of rocky intertidal habitats by juvenile pollock Pollachius virens. Marine Ecology Progress Series, 126: 9–17.
Rebours C, Marinho-Soriano E, Zertuche-González JA, Hayashi L, Vásquez JA, Kradolfer P, et al. 2014. Seaweeds: an opportunity for wealth and sustainable livelihood for coastal communities. Journal of Applied Phycology, 26: 1939–1951.
Reynolds RW, Smith TM, Liu C, Chelton DB, Casey KS, and Schlax MG. 2007. Daily high-resolution-blended analyses for sea surface temperature. Journal of Climate, 20: 5473–5496.
Schmidt AL, Coll M, Romanuk TM, and Lotze HK. 2011. Ecosystem structure and services in eelgrass Zostera marina and rockweed Ascophyllum nodosum habitats. Marine Ecology Progress Series, 437: 51–68.
Schonbeck M, and Norton TA. 1978. Factors controlling the upper limits of fucoid algae on the shore. Journal of Experimental Marine Biology and Ecology, 31(3): 303–313.
Sears JR. (Ed.). 2002. NEAS keys to benthic marine algae of the northeastern coast of North America from Long Island Sound to the Strait of Belle Isle. 2nd edition. Express Printing, Fall River, Massachusetts. NEAS Contribution Number 2.
Seeley RH, and Schlesinger WH. 2012. Sustainable seaweed cutting? The rockweed (Ascophyllum nodosum) industry of Maine and the Maritime Provinces. Annals of the New York Academy of Sciences, 1249: 84–103.
Smale DA, Burrows MT, Moore P, O’Connor N, and Hawkins SJ. 2013. Threats and knowledge gaps for ecosystem services provided by kelp forests: a northeast Atlantic perspective. Ecology and Evolution, 3(11): 4016–4038.
Steneck RS, Graham MH, Bourque BJ, Corbett D, Erlandson JM, Estes JA, et al. 2002. Kelp forest ecosystems: biodiversity, stability, resilience and future. Environmental Conservation, 29(04): 436–459.
Tanaka K, Taino S, Haraguchi H, Prendergast G, and Hiraoka M. 2012. Warming off southwestern Japan linked to distributional shifts of subtidal canopy-forming seaweeds. Ecology and Evolution, 2(11): 2854–2865.
Taylor WR. 1957. Marine algae of the northeastern coast of North America. University of Michigan Press, Ann Arbor, Michigan. 509 p.
Torossian JL, Kordas RL, and Helmuth B. 2016. Chapter Eight—Cross-scale approaches to forecasting biogeographic responses to climate change. Advances in Ecological Research, 55: 371–433.
Tuya F, Cacabelos E, Duarte P, Jacinto D, Castro JJ, Silva T, et al. 2012. Patterns of landscape and assemblage structure along a latitudinal gradient in ocean climate. Marine Ecology Progress Series, 466: 9–19.
Ugarte R, and Sharp G. 2012. Management and production of the brown algae Ascophyllum nodosum in the Canadian maritimes. Journal of Applied Phycology, 24: 409–416.
Van Guelpen L, Chmura GL, and Pohle G. 2007. Climate change and thermal sensitivity of Canadian Atlantic commercial marine species. Report to the Climate Change Impacts and Adaptation Program. Natural Resources Canada for Agreements A515 and A515a, Natural Resources Canada, Ottawa, Ontario.
Walther G-R, Post E, Convey P, Menzel A, Parmesan C, Beebee TJC, et al. 2002. Ecological responses to recent climate change. Nature, 416(6879): 389–395.
Watling JI, Bucklin DN, Speroterra C, Brandt LA, Mazzotti FJ, and Romañach SS. 2013. Validating predictions from climate envelope models. PLoS ONE, 8(5): e63600.
Wernberg T, Russell BD, Thomsen MS, Gurgel CFD, Bradshaw CJA, Poloczanska ES, et al. 2011. Seaweed communities in retreat from ocean warming. Current Biology, 21(21): 1828–1832.
Wikström SA, and Kautsky L. 2007. Structure and diversity of invertebrate communities in the presence and absence of canopy-forming Fucus vesiculosus in the Baltic Sea. Estuarine, Coastal and Shelf Science, 72: 168–176.
Wilson KL, Kay LM, Schmidt AL, and Lotze HK. 2015. Effects of increasing water temperatures on survival and growth of ecologically and economically important seaweeds in Atlantic Canada: implications for climate change. Marine Biology, 162: 0.
Information & Authors
Information
Published In
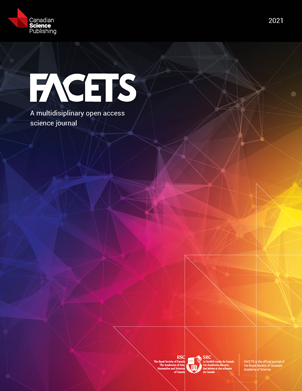
FACETS
Volume 3 • Number 1 • October 2018
Pages: 275 - 286
Editor: Daniel E. Schindler
History
Received: 30 June 2017
Accepted: 3 November 2017
Version of record online: 12 March 2018
Copyright
© 2018 Khan et al. This work is licensed under a Creative Commons Attribution 4.0 International License (CC BY 4.0), which permits unrestricted use, distribution, and reproduction in any medium, provided the original author(s) and source are credited.
Data Availability Statement
All relevant data are within the paper and available from the sources mentioned in the Methods section.
Key Words
Sections
Subjects
Plain Language Summary
Warming oceans threaten commercially harvested seaweeds in Atlantic Canada
Authors
Author Contributions
LVG, GP, and GLC conceived and designed the study.
AHK, LVG, and GP performed the experiments/collected the data.
AHK, EL, and GLC analyzed and interpreted the data.
EL and GLC contributed resources.
AHK, EL, and GLC drafted or revised the manuscript.
Competing Interests
The authors have declared that no competing interests exist.
Metrics & Citations
Metrics
Other Metrics
Citations
Cite As
Amina H. Khan, Elisabeth Levac, Lou Van Guelphen, Gerhard Pohle, and Gail L. Chmura. 2018. The effect of global climate change on the future distribution of economically important macroalgae (seaweeds) in the northwest Atlantic. FACETS.
3(1): 275-286. https://doi.org/10.1139/facets-2017-0091
Export Citations
If you have the appropriate software installed, you can download article citation data to the citation manager of your choice. Simply select your manager software from the list below and click Download.
Cited by
1. Origins and pathways of the floating Sargassum in the Yellow and East China Seas
2.
3.
4. A field study of the molecular response of brown macroalgae to heavy metal exposure: An (epi)genetic approach
5. Subarctic sugar kelp (
Saccharina latissima
, Phaeophyceae) summer productivity and contribution to carbon budgets
6. Secular change in seaweed species composition and coverage of Sargassaceae on the artificial reef in Wakasa Bay, Japan
7. Blue carbon assessment in Avicennia marina sediments and vegetation along the Red Sea Coast of Egypt: Improving methods and insights
8. Secular change in seaweed species composition and coverage of Sargassaceae on the artificial reef in Wakasa Bay, Japan
9. What if there is no further south to go: Assessing the vulnerability of Nacella species to climate change
10. Livestock feed resources used as alternatives during feed shortages and their impact on the environment and ruminant performance in West Africa: a systematic review
11. Functioning of a canopy-dominated intertidal community during emersion: highly productive but heterotrophic at the annual scale
12. Technological innovation in bioformulation between algae and PGPR to optimize germination and early growth of horticultural species
13. Projecting environmental suitability areas for the seaweed Gracilaria birdiae (Rhodophyta) in Brazil: Implications for the aquaculture pertaining to five environmentally crucial parameters
14. Biodiversity and biogeography of microalgae with food and feed potential
15. Effects of Global Warming on the Growth and Proliferation of Attached Sargassum horneri in the Aquaculture Area near Gouqi Island, China
16. Gaps in knowledge about distribution, utilization, and management of Sargassaceae (Fucales, Phaeophyta) in Okinawa Island, southern Japan
17. Tracking the effect of climatic and non-climatic elements on rice production in Pakistan using the ARDL approach
18. A spatially-modelled snapshot of future marine macroalgal assemblages in southern Europe: Towards a broader Mediterranean region?
19. Modeling the Growth Potential of the Kelp Saccharina Latissima in the North Atlantic
20. Sustainable resource production for manufacturing bioactives from micro‐ and macroalgae: Examples from harvesting and cultivation in the Nordic region
21. Application of omics research in seaweeds with a focus on red seaweeds
22. Effects of Temperature and Light on Growth Rate and Photosynthetic Characteristics of Sargassum horneri
23. Effects of ocean warming, eutrophication and salinity variations on the growth of habitat-forming macroalgae in estuarine environments
24. Ecological performance differs between range centre and trailing edge populations of a cold-water kelp: implications for estimating net primary productivity
25. Latitudinal and Seasonal Changes in Intertidal Sea Surface Temperature Along the Atlantic Coast of Nova Scotia, Canada
26. Sustainable management of economically valuable seaweed stocks at the limits of their range of distribution: Ascophyllum nodosum (Phaeophyceae) and its southernmost population in Europe
27. Seaweed resources, collection, and cultivation with respect to sustainability
28. Predictions of kelp distribution shifts along the northern coast of Japan
29. A review of current uses and potential biotechnological applications of seaweeds from the Macaronesian region (Central-East Atlantic Ocean)
30. Projecting marine species range shifts from only temperature can mask climate vulnerability
31. Evidence for different thermal ecotypes in range centre and trailing edge kelp populations