Exaggerated expectations in ancient starch research and the need for new taphonomic and authenticity criteria
Abstract
Ancient starch research illuminates aspects of human ecology and economic botany that drove human evolution and cultural complexity over time, with a special emphasis on past technology, diet, health, and adaptation to changing environments and socio-economic systems. However, lapses in prevailing starch research demonstrate the exaggerated expectations for the field that have been generated over the last few decades. This includes an absence of explanation for the millennial-scale survivability of a biochemically degradable polymer, and difficulties in establishing authenticity and taxonomic identification. This paper outlines new taphonomic and authenticity criteria to guide future work toward designing research programs that fully exploit the potential of ancient starch while considering growing demands from readers, editors, and reviewers that look for objective compositional identification of putatively ancient starch granules.
Introduction
Ugent et al. (1982) published the earliest account of archaeological starch granules from work at sites north of Lima (Peru) more than 35 years ago. Recovered granules from 4000-year-old desiccated tubers were identified as potato starch using optical and histological techniques. The authors attributed the exceptional preservation of starch to extreme aridity, and used granule morphology to infer source plant taxonomy, a practice with origins in late 19th and early 20th century biochemistry (Nageli and Nageli 1858; Meyer 1895; Reichert 1913). This approach has persisted until today (Czaja 1978; Jane et al. 1994; Torrence and Barton 2006). During the ensuing decades, the pioneering combination of starch granule identification and studies of early human diets opened the door for an exponential growth of the field and publicity in high impact venues (e.g., Loy et al. 1992; Piperno et al. 2000, 2004, 2009; Perry et al. 2006, 2007; Mercader 2009). These studies focused mainly on sites from the Americas and Australasia, and established the extraction, detection, description, and identification methods currently in use. Since then, dozens of articles have appeared in the field of archaeology on the pervasive, but little understood, presence of starch recovered from tools, sediments, and recently, dental calculus (Henry and Piperno 2008, 2011; Hardy et al. 2009, 2012, 2016; Salazar-García et al. 2013).
New taphonomic criteria and authentication
Overall, the need for revision stems from multiple challenges common to the discipline including:
1.
small recovered assemblages,
2.
high contamination risk in both the field (Hart 2011; Laurence et al. 2011; Dozier 2016; Mercader et al. 2017) and the laboratory (Loy and Barton 2006; Crowther et al. 2014; García-Granero et al. 2016),
3.
poor understanding of how plant-derived biomolecules and their often complex diagenetic products adsorb onto surfaces,
4.
disparity in reporting standards,
5.
scarcity of systematically controlled experimentation,
6.
lack of a plausible explanation for the millennial-scale survivability of a biochemically degradable polymer,
7.
difficulty in authentication, and
8.
uncertainty surrounding taxonomic identification (Copeland and Hardy 2018; Mercader et al. 2018a, 2018b).
These gaps in the prevailing starch research perpetuate skepticism and generate inflated notions. This paper is a roadmap organised around two of the aforementioned challenges: taphonomic pathways and authentication, even though there are no standard criteria to address these challenges. This paper provides examples of experimental research that may lead to formal sets of criteria in the near future.
Fresh starch granules are polysaccharide polymers with semi-crystalline and amorphous regions, exhibiting unmodified birefringence from a concentric arrangement of crystalline, helical amylopectin clusters. Customarily, in archaeology, confirmation of starch chemistry uses polarised light microscopy of birefringent granules to display a distinctive “Maltese cross”. The biosynthetic deposition of the crystalline starch granules occurs within plant organelles inside cells surrounded by lignocellulosic walls. When the cells decay, a few of the starch granules may be afforded physical protection through entanglement in the remnant cell wall matrix. Commonly, starch granules do not exist in isolation; they are admixed with non-starch polysaccharides, lipids, and proteins co-occurring in variable proportions, depending on the plant source. Chemical reactions with these co-concurrent molecules must be taken into account when attempting to conceptualise diagenetic processes of starch granules in archaeological contexts. Starch is a highly accessible biological energy reserve and the second most ubiquitous polysaccharide after the structural form known as cellulose. Unfortunately, as with many other biopolymers, starch is highly susceptible to hydrolysis over long timescales and the survival of these molecules only occurs under special circumstances (Briggs et al. 2000). The fate of plant biomarkers depends on what happens to the molecule immediately after it is released into the environment (Butts and Briggs 2011), and it is in the early stages of burial that the potential for degradation is greatest. A starch rich plant fragment can contain billions of starch granules, yet can these retain sufficient biochemical functionality and morphology to be identified as starch related thousands of years later?
It can be hypothesised that surfaces where starch granules become enzymatically unavailable, adsorbed to minerals, coated, overgrown, entrapped, or protected from chemical degradation, would be potentially rich locations at which to look for ancient starches (Box 1). Based on existing literature and ongoing experimentation, we outline six potential taphonomic pathways currently being investigated that hold promise for better understanding starch preservation or degradation in common archaeological contexts such as stone tools and dental calculus.
Box 1. Taphonomic pathways.
•
Sorption
•
Enzymatic inaccessibility
•
Microbial modulation
•
Silicification
•
Phosphatisation
•
Non-enzymatic breakdown
Sorption to mineral phases
Adherence and bonding to solid substrates is governed by chemical sorption and by previously adsorbed molecules and functional groups (Collins et al. 1995; Kislenko 2002; Demarchi et al. 2016). In this scenario, adsorption delivers a relatively strong bond between the polymer and, for example, the mineral surface of a stone tool. The presence of oxides or other weathering products, surface roughness, pH, and complexation of species through ligand exchange could mediate binding (Dowell 1985; McKnight et al. 1992; Kaiser and Guggenberger 2000). It can be surmised that if starch were to become encapsulated in a mineral phase due to precipitation of the mineral and (or) recrystallisation, this would result in neoformed minerals and micro-structural transformations (e.g., a granule coating) that confer visual properties under polarised light (Lee-Thorp 2002; Briggs 2003) that are clearly distinct from those in native starch. Mineralised granules diffract polarised light in a mosaic pattern that combines strong birefringence (first order crystallisation) with disrupted, isotropic zones (J. Mercader, personal observation, 2017).
Enzymatic bioavailability
There is vast literature on the mechanism of starch hydrolysis by enzymes under variable water and temperature conditions (Hoover 2000; Singh et al. 2010; Wang and Copeland 2015). Other studies have reviewed the process of gelatinisation due to its central role in food processing (Hoover 2000; Ratnayake and Jackson 2008; Wang and Copeland 2013; Wang et al. 2017). Hydration mobilises glucose and makes starch highly reactive to molecular degradation and susceptible to consumption by microbiota demonstrating that access to the glucosidic bonds inside the granules is the key to preservation. Mobility and activity of hydrolytic enzymes are limited under low water and temperature conditions, as thermal states permitting gelatinisation allow water molecule diffusion into amylose and amylopectin chains, whereas temperatures below gelatinisation retard water ingress. Water, however, can still move inside granules at low temperatures, for example, in a process known as annealing (Jacobs and Delcour 1998).
Much has been written about archaeological starch granules existing in the sheltered niche of a microscopic artefact crevice (Piperno et al. 2000; Barton and Matthews 2006; Pearsall 2015). This scenario, however, has weak empirical support. Many groups of microorganisms inhabit rock surfaces and form lithobiontic coatings (Dorn 1998), which further support colonisation of the stone’s surface, fractures, and pores by bacteria, fungi, and lichen. Many alpha-amylases are exoenzymes (i.e., they are exported out of the cell and work outside the cell). These are much smaller than cells. The smallest known bacteria are 200 nm in diameter (Luef et al. 2015), whereas alpha-amylases range from 40–160 kDa, equivalent to approximately 4–8 nm in diameter (Mehta and Satyanarayana 2016). Therefore, only the enzyme would need to enter a crevice for colonisation, down to a scale of hundreds of nanometers. Moreover, organic material and water commonly co-occur in stone crevices, further accumulating and attracting fungal and bacterial communities to interstitial spaces and microfractures (Golubic and Schneider 2003). Therefore, crevices alone are unlikely to offer protection to starch from hydration or decay, and could, in fact, promote starch degradation.
Research surrounding starch resistance to degradation has seldom been at the forefront of soil and sedimentary analysis. The persistence of carbohydrates in soils, sediments, and surfaces may be due to enzymatic inaccessibility (Cheshire et al. 1969; Cheshire 1977; Dungait et al. 2012). Salts may affect gelatinisation (Gough and Pybus 1973; Jane 1993; Zhu et al. 2009; García-Díaz et al. 2016; Wang et al. 2017), with starch granules close to mineral surfaces possibly having reduced susceptibility to gelatinisation. The chemical and enzymatic lability of organic matter in soils is related to temperature, mineral surface area, encapsulation, heterogeneity, and oxygen (Baldock and Skjemstad 2000; Lützow et al. 2006; Schmidt et al. 2011). Furthermore, laboratory studies show that the rate of enzymatic degradation of starch is affected by molecular organisation (Tester et al. 2006), hydration (Kayisu and Hood 1979), the optimum pH for bacterial and fungal amylases (Pandey et al. 2000; Saranraj and Stella 2013), the presence of Na+, Ca2+, and Mg2+ salts that impact amylase production (Gupta et al. 2003), and retrogradation. Physico-chemical differences between starches, including variable crystallography, may be another key to understanding the variance in the survivability of the starch polymer (Park et al. 2007).
Microbial degradation cycles
Despite the archaeological emphasis in studying the geochemical conditions for starch degradation in soils and sediments, little is known about the tempo and mode of microbial decay of starch (Adu and Oades 1978) and the possibility of biofilm-mediated mineralisation (Pacton et al. 2007). Observations over the course of several months, using a diversity of matrices over a range of pH conditions, confirmed the microbial degradation of starch in cycles, where the microbiome clades in the model system dominated hydrolysis at the expense of other clades from the soil itself (P. Dunfield, J. Kim, J. Mercader, personal observation, 2017; Fig. 1, Table 1). This initial observation prompted further experimentation by PD, TA, and JM, whereby soil microbiota under various moisture levels and oxygen conditions hydrolyse different starches. These microcosms are being sampled at regular intervals to study the microbial metabolic activity through gas chromatography, identify the presence or absence of various microbial clades involved in starch degradation through DNA analysis, and record the morphological changes that bacteria trigger in starch granules during alteration under the microscope.
Fig. 1.

Table 1.
Hypoxis sample mass (g) | Experiment type | Substratum | pH | Inoculum |
---|---|---|---|---|
0.3 | Single variable | Nanospheres + clay (autoclaved) | 5 | Trichoderma |
0.3 | Single variable | Nanospheres + clay (autoclaved) | 7 | Bacillus |
0.3 | Single variable | Nanospheres + clay (autoclaved) | 5 | African soil mixed medium |
0.3 | Single variable | Nanospheres + clay (autoclaved) | 7 | African soil mixed medium |
0.3 | Single variable | Nanospheres + clay (autoclaved) | 10 | African soil mixed medium |
0.3 | Control | African soil (natural) | n/a | n/a |
0.3 | Control | African soil (autoclaved) | n/a | African soil mixed medium |
0.3 | Control | Nanospheres + clay (autoclaved) | n/a | n/a |
A promising model to distinguish the effects of microbial activity on starch granules is found in studies of modern soil surfaces (Haslam 2004; Hutschenreuther et al. 2017; Mercader et al. 2017), where scientists can study and quantify the effects of soil microbes on starch granule shape, size, surface texture, and crystallinity in vivo. Bacterial “preference” for starches from certain plant taxa suggests that the differential survivability of starches is likely to bias archaeological starch records (Hutschenreuther et al. 2017). A recent study of Tanzanian soil surfaces (Mercader et al. 2017) confirms the impact microbial degradation has on starch architecture in short periods, with granule populations displaying pitting, clefting, slitting, implosion of the hilum, fissuring, and most importantly, pervasive crystallite disruption that results in partial or complete loss of granule birefringence under cross-polarised light. These modifications present us with a problem because the changes inflicted on the granule by natural damage, especially over thousands of years, can overlap with those caused by culinary modification (Henry et al. 2010; Collins and Copeland 2011; Crowther 2012).
Silicification
The term “microfossil” has pervaded the literature of ancient starch research, even though there is no evidence that any archaeological starch granule reported in the literature is actually fossilised, unlike palaeontological analogs (Baxter 1964; Wilkinson 1983; Locatelli 2014). The term “exceptional preservation” refers to a fossilisation mode that preserves fragile structures but not their original biochemical features. However, even in cases where exceptional preservation is invoked, any presumption that a microfossil is in its original, unaltered biological form needs to be testable given the generally improbable nature of biological molecule preservation over long time scales (Schweitzer 2011). Normally, ancient and modern molecular counterparts display significant architectural and elemental differences (Gupta and Briggs 2011). During burial, the shielding of labile molecules from complete destruction can take place through aliphatic structural enrichment in the preserved organic matter, a product of diagenesis and kerogen formation (Cody et al. 2011), which results in hydrophobicity (Gupta and Pancost 2004) and chemically resistant, neoformed geo-macromolecules (Gupta et al. 2009).
Fossilisation of starch granules is feasible through the silicification of materials adhered to surfaces when, after burial, they could be permeated by interstitial waters rich in solute minerals that precipitate during wetting and drying cycles. Silicified macrobotanicals are common at famous archaeological localities, such as Olduvai Gorge (Tanzania) (Bamford 2012), and provide independent evidence of silicification as an active fossil forming process in burial environments of interest to archaeologists. Decades of experimental fossilisation studies (Drum 1968; Briggs and Kear 1993; Channing and Edwards 2004; Townson et al. 2014) demonstrate that plant parts permeated by silica-rich solutions undergo fast silicification by the deposition of opaline silica on polysaccharide surfaces and within voids, ideal for mineral nucleation sites (cf. Kealhofer et al. 1999; Smith and Stockey 2002; Butts and Briggs 2011). Under controlled laboratory conditions, authigenic mineralisation of potato, wheat, and corn starch occur by percolating them with sodium silicate, magnesium silicate (Wollast et al. 1968), or colloidal silica solutions (Oehler and Schopf 1971) under different water activity, heat, and pressure regimes over the course of several weeks produced variable silicification modes (J. Mercader, M. Soto, J. Inwood, personal observation, 2018; Fig. 2). Often, the resulting mineralisations have fully polymerised with silica and have disrupted the native amylopectin crystallites, as shown by neoformed crystallisations with strong 1st and 2nd order birefringence and complete disappearance of the “Maltese cross”. These efforts suggest that starch granule morphology can be preserved through such mineral deposition processes.
Fig. 2.

Phosphatisation of starch granules in dental plaque
There remain many unsolved questions surrounding the entrapment, co-precipitation, cementation, coating, and mineralisation of starch granules in dental calculus, which is a biogenic calcium phosphate precipitate that often contains microbotanical remains (e.g., Armitage 1975; Fox et al. 1996; Hayashizaki et al. 2008; Hardy et al. 2009; Lee et al. 2013; Power et al. 2014). To understand the potential for the survivability of starch granules from plaque to various aggressors we observed the co-precipitation of calcium phosphate and starch to study artificial calculus under controlled conditions. By replicating calculus formation, we discovered that potato starches can endure high levels of oral amylase and appear to be shielded from the gelatinising effect of sodium hydroxide (Fig. 3), presumably after mineral exchange with the phosphatising environment has permeated the starch granule surface. This indicates that entombment of organic matter in a calcium phosphate environment has the potential to transform, delay, or even completely suppress microbial action on organic remains (Briggs and Summons 2014). Ongoing studies to build model calculus onto communities of living oral microbiota will further elucidate the manner in which starches become entrapped and protected from amylases and other diagenetic agents.
Fig. 3.

Starch breakdown and byproduct preservation
The chemical properties of edible starches, including attributes such as lipid, moisture and ash content, amylose:amylopectin ratio, crystallinity (as indicated by the X-ray diffraction pattern), and structural characteristics have been extensively documented (Hoover 2000; Ancona et al. 2011; Olayinka et al. 2013). To an archaeologist, this information is invaluable for understanding how these molecules react to the process of degradation in taphonomic contexts. It is imperative that these characterisations be carried out on species of archaeological interest to identify differences in breakdown pathways, and to aid in biomarker identification and the potential neoformation of high molecular mass species (Gupta et al. 2007; Gupta 2015) or other signature recalcitrant products.
In addition to the enzymatic degradation of starch, we must consider non-enzymatic interactions between proteins and starches and their degradation products as a potential route to producing refractory species. The Maillard reaction, the abiotic reaction of amino acid and carbohydrate species, produces complex chemistries proposed as one of the routes of preservation of organic matter in soils and kerogens (Larter and Douglas 1980). This reaction, which occurs under ambient conditions and at high temperatures during cooking, produces a variety of high molecular mass pigmented products or “melanoidins” (Ajandouz and Puigserver 1999; Hill et al. 2005; Hwang et al. 2011). Figure 4 shows the results of a Fourier transform ion cyclotron resonance mass spectrometry (FTICR-MS) study. The model melanoidin system was generated by the reaction of glycine and wheat starch in water heated for 64 h at 150 °C and 200 °C, respectively. Although the reaction was carried out at unrealistic elevated temperatures to promote rapid reaction, the analysis showed that a diverse range of cyclic and multiple heteroatoms are easily produced from simple chemical precursors (Erickson 2009). In addition, an increase in the temperature of the Maillard reaction shows a loss in higher mass species and reduction in the number of heteroatoms per molecule yielding to more recalcitrant products. Although the environmental kinetics and relevance of the so-called Maillard reaction under archaeological conditions are yet to be fully established, the ready conversion of biologically accessible species to more refractory ones suggests that this reaction is worthy of investigation as a route to preserving starch-related carbon.
Fig. 4.

Authentication procedures
The notion that a semi-crystalline organic polymer such as starch could be preserved and remain undamaged for hundreds or thousands of years is contrary to chemical and taphonomic assumptions. Thus, claims of residual starch in deep time environments demand solid, multidisciplinary evidence. Authentication procedures should be multipronged (Box 2). Above, we have proposed several taphonomic pathways that may lead to a better understanding of how ancient starch may survive destruction. If mineralisation, chemical attack, microbial degradation, and recrystallisation leave a taphonomic imprint that, optically speaking, separates these preserved granules from their fresh counterparts, what is required in future research is additional analyses encompassing micromorphology, elemental composition, and molecular chemical structure to tease out compositional differences that may exist in putative ancient granules relative to reference materials.
Box 2. Authentication traits and procedures.
•
Microstructure
•
Molecular architecture
•
Elemental composition
•
Position specific isotopes
•
Excavation in decontaminated spaces
•
Lab processing of samples in cleanrooms
Techniques that can achieve elemental and structural characterisation of discrete starch granule surfaces and microtomed specimens include:
•
Raman and Fourier transform infrared spectroscopy,
•
energy dispersive X-rays from scanning/transmission electron microscopy (SEM/TEM).
Raman spectroscopy can photo-bleach discreet target granules and therefore detect characteristic G and D bands from amorphous carbon. In addition, performing Raman spectroscopy on microtomed granules can illustrate vibration regions that highlight differences in molecular structure between native and fossil starches. SEM/TEM can reveal features commonly seen in starch such as damaged blocklets and endocorrosion pits (Pérez et al. 2009) as well as hydrolysed shells with channels (Huang et al. 2014). The melting of crystallites and recrystallisation microstructures can also be detected in a granule’s cross section by SEM.
Atomic force microscopy is widely used as a technique to probe the surface of starch granules (Gallant et al. 1997; Baldwin et al. 1998; Dang and Copeland 2003). However, degradation products may pose challenges for effective analysis, as the resolution of this technique requires that samples be extremely clean and free from coatings. It is also worth exploring the new generation of high-resolution coupled imaging and mass spectrometry tools such as secondary ion mass spectrometry (SIMS).
It remains problematic to excavate archaeological artefacts under field conditions that cannot assure the systematic avoidance of contamination (Laurence et al. 2011). Mercader et al. (2017) recommended that field archaeologists characterise the starch contamination landscape that is specific to their study area and utilise dedicated excavation tools that can be cleaned frequently with a solvent or starch gelatinising agent. It can be beneficial to wear disposable clean attire in the excavation area, isolate excavation areas from excessive traffic, and use gloves and sample bags (confirmed to be starch-free) for contact with artefacts. Once retrieval and storage compromise samples, it can be challenging to separate target and contaminant starches. Commonly used decontaminating methods such as airbrushing by compressed air and mild washing (Barton and Torrence 2015) can fail to remove recent intrusions even after several ultrasonic cleaning cycles (Pedergnana et al. 2016; Cnuts and Rots 2017; Mercader et al. 2017).
Regarding ancient contamination, functional analysis via use-wear studies and spatial sampling of residues have traditionally buttressed the interpretation of starch granules as genuinely related to ancient utilisation, if it has been demonstrated that the location of the starches was along the used edges of archaeological tools. Unfortunately, recent work has also called this link into question (Rots et al. 2016), as the location of residues does not necessarily indicate that they are use-related (Xhauflair et al. 2017) or contamination distributed along edges (Pedergnana and Ollé 2017). New experimental research (J. Mercader, M. Soto, personal observation, 2018) is focusing on detecting patterns of microbotanical attachment in natural rocks (lying on modern surfaces and in contact with surrounding soils) to build a baseline whereby residue density estimation by geographic information system (GIS) and nearest neighbour heat maps of plant materials adhering to rock surfaces can be compared with residue scatters from stone tools. Emerging results indicate that naturally attached plant matter consistently presents a dispersed pattern (Fig. 5).
Fig. 5.

Anti-contamination laboratory protocols remain underdeveloped, under-utilised, and under-reported (Crowther et al. 2014), prompting immediate attention. Contaminant starches are a threat and subpar testing techniques for environmental contamination (e.g., small numbers of passive traps) severely underestimate the magnitude of the problem. It should not be assumed that consumables are starch-free, even when laboratory suppliers state so. The potential for unfiltered, recirculated air to propel large numbers of starch particles around a lab volume has been confirmed (Crowther et al. 2014). It is worth re-evaluating whether archaeological assemblages consisting of small numbers of starch granules could be a false positive. Importantly, this concern about the integrity of the evidence pertains to assemblages derived from all kinds of archaeological materials, including stone tools, dental calculus, sediments, and pottery surfaces.
Conclusions
Although ancient starch researchers and archaeological residue analysts have reflected on the extraordinary biological, geological, and chemical circumstances that must have occurred if such a fragile biopolymer were to survive on a millennial scale (Barton 2009; Henry 2015), there has been little progress toward characterising starchy materials compositionally or directly investigating the taphonomic and diagenetic challenges for the persistence of starch granules chemically and morphologically. We need a new biogeochemical paradigm (Box 3) to understand changes in deposited starch over time that addresses poorly understood processes of degradation, recrystallisation, and (or) permineralisation. The morphological preservation of a granular structure does not imply the chemical conservation of starch. We recognise starch through imaging techniques because of its crystallinity. After degradation, how do we detect its altered states if not through optical behaviour? Future work must consider growing demands from readers, editors, and reviewers that look for chemical identification of presumed starch granules and, thus, the need for verified chemical characterisation techniques to secure reliable molecular fingerprints from optically targeted sample sites. Experimentation and theoretical modelling from chemical computation (Damm et al. 1997; Molinero and Goddard 2004; Limbach and Kremer 2006; Fadda and Woods 2010) could help us understand starch degradation over thousands of years. New limits can be explored, and multidisciplinary collaboration with biology, geoscience, and chemistry can help deliver a set of best practices in which data acquisition, authentication, analysis, reporting, and data sharing could lead to realising the full potential (Fenn and Raskino 2008) of a controversial archaeological technique that still holds great promise to shed light on myriad aspects of the past.
Box 3. Examples of ongoing efforts.
•
Research to assess the impact of microorganisms on starch hydrolysis (Fig. 1)
•
Research to understand the silicification of starches in silica-rich environments (Fig. 2)
•
Research to examine mineral transfer between starches and artificial dental plaque (Fig. 3)
•
Research to evaluate the production and survivability of compound classes in a Maillard reaction of starch and amino acids (Fig. 4)
•
Research to investigate the pattern of residue adherence to natural rocks to distinguish them from anthropogenic residues (Fig. 5).
Acknowledgements
This paper was inspired by discussions at a workshop titled “Ancient Starch Research: Goals, Pitfalls, Possibilities” held in Zanzibar, Tanzania, 15–16 June 2017. This event was fully sponsored by the Canadian Social Sciences and Humanities Research Council under its Partnership Grant Program no. 895-2016-1017. We thank four anonymous reviewers, the FACETS editorial team, Dr. Tom Al, Dr. Shah Nilar for suggestions on the scope of theoretical simulations, and Siobhán Clarke, whose constructive comments greatly improved the quality of the manuscript.
References
Adu JK, and Oades JM. 1978. Utilization of organic materials in soil aggregates by bacteria and fungi. Soil Biology and Biochemistry, 10(2): 117–122.
Ajandouz EH, and Puigserver A. 1999. Nonenzymatic browning reaction of essential amino acids: effect of pH on caramelization and Maillard reaction kinetics. Journal of Agricultural and Food Chemistry, 47(5): 1786–1793.
Ancona DB, Campos MRS, Guerrero LAC, and Ortíz GD. 2011. Structural and some nutritional characteristics of Velvet bean (Mucuna pruriens) and Lima bean (Phaseolus lunatus) starches. Starch/Stärke, 63(8): 475–484.
Armitage PL. 1975. The extraction and identification of opal phytoliths from the teeth of ungulates. Journal of Archaeological Science, 2(3): 187–197.
Baldock JA, and Skjemstad JO. 2000. Role of the soil matrix and minerals in protecting natural organic materials against biological attack. Organic Geochemistry, 31(7–8): 697–710.
Baldwin PM, Adler J, Davies MC, and Melia CD. 1998. High resolution imaging of starch granule surfaces by atomic force microscopy. Journal of Cereal Science, 27: 255–265.
Bamford MK. 2012. Fossil sedges, macroplants, and roots from Olduvai Gorge, Tanzania. Journal of Human Evolution, 63(2): 351–363.
Barton H. 2009. Starch granule taphonomy: the results of a two year field experiment. In Archaeological science under a microscope: studies in residue and ancient DNA analysis in honour of Thomas H. Loy. Edited by M Haslam, G Robertson, A Crowther, S Nugent, and L Kirkwood. ANU E Press, Canberra, Australia. pp. 129–140.
Barton H, and Matthews PJ. 2006. Taphonomy. In Ancient starch research. Edited by R Torrence and H Barton. Left Coast Press, Walnut Creek, California. pp. 75–94.
Barton H, and Torrence R. 2015. Cooking up recipes for ancient starch: assessing current methodologies and looking to the future. Journal of Archaeological Science, 56: 194–201.
Baxter RW. 1964. Paleozoic starch in fossil seeds from Kansas coal balls. Transactions of the Kansas Academy of Science, 67(3): 418–422.
Briggs DEG. 2003. The role of decay and mineralization in the preservation of soft-bodied fossils. Annual Review of Earth and Planetary Sciences, 31: 275–301.
Briggs DEG, and Kear AJ. 1993. Fossilization of soft-tissue in the laboratory. Science, 259: 1439–1442.
Briggs DEG, and Summons RE. 2014. Ancient biomolecules: their origins, fossilization, and role in revealing the history of life. BioEssays, 36(5): 482–490.
Briggs DEG, Evershed RP, and Lockheart MJ. 2000. The biomolecular paleontology of continental fossils. The Paleontological Society, 26(4): 169–193.
Butts SH, and Briggs DEG. 2011. Silicification through time. In Taphonomy: aims & scope topics in geobiology. Edited by PA Allison and DJ Bottjer. Springer, Dordrecht, South Holland, the Netherlands. pp. 411–434.
Channing A, and Edwards D. 2004. Experimental taphonomy: silicification of plants in Yellowstone hot-spring environments. Transactions of the Royal Society of Edinburgh: Earth Sciences, 94: 503–521.
Cheshire MV. 1977. Origins and stability of soil polysaccharide. European Journal of Soil Science, 28(1): 1–10.
Cheshire MV, Mundie CM, and Shepherd H. 1969. Transformation of 14C glucose and starch in soil. Soil Biology and Biochemisty, 1(2): 117–130.
Cnuts D, and Rots V. 2017. Extracting residues from stone tools for optical analysis: towards an experiment-based protocol. Archaeological and Anthropological Sciences, 1–20.
Cody GD, Gupta NS, Briggs DE, Kilcoyne ALD, Summons RE, Kenig F, et al. 2011. Molecular signature of chitin-protein complex in Paleozoic arthropods. Geology, 39(3): 255–258.
Collins MJ, and Copeland L. 2011. Ancient starch: cooked or just old? Proceedings of the National Academy of Sciences of the USA, 108(22): E145.
Collins MJ, Bishop AN, and Farrimond P. 1995. Sorption by mineral surfaces: rebirth of the classical condensation pathway for kerogen formation? Geochimica et Cosmochimic Acta, 59(11): 2387–2391.
Copeland L, and Hardy K. 2018. Archaeological starch. Agronomy, 8(1): 4.
Crowther A. 2012. The differential survival of native starch during cooking and implications for archaeological analyses: a review. Archaeological and Anthropological Sciences, 4(3): 221–235.
Crowther A, Haslam M, Oakden N, Walde D, and Mercader J. 2014. Documenting contamination in ancient starch laboratories. Journal of Archaeological Science, 49: 90–104.
Czaja AT. 1978. Structure of starch grains and the classification of vascular plant families. Taxon, 27(5/6): 463–470.
Damm W, Frontera A, Tirado-Rives J, and Jorgensen WL. 1997. OPLS all-atom force field for carbohydrates. Journal of Computational Chemistry, 18(16): 1955–1970.
Dang JMC, and Copeland L. 2003. Imaging rice grains using atomic force microscopy. Journal of Cereal Science, 37: 165–170.
Demarchi B, Hall S, Roncal-Herrero T, Freeman CL, Woolley J, Crisp MK, et al. 2016. Protein sequences bound to mineral surfaces persist into deep time. eLife, 5: e17092.
Dorn RI. 1998. Rock coatings. Elsevier, New York City, New York. 429 p.
Dowell WHM. 1985. Kinetics and mechanisms of dissolved organic carbon retention in a headwater stream. Biogeochemistry, 1(4): 329–352.
Dozier CA. 2016. Airborne starch dispersal from stone grinding: experimental results and implications. Journal of Archaeological Science: Reports, 8: 112–115.
Drum RW. 1968. Silicification of Betula woody tissue in vitro. Science, 161(3837): 175–176.
Dungait JAJ, Hopking DW, Gregory AS, and Whitmore AP. 2012. Soil organic matter turnover is governed by accessibility not recalcitrance. Global Change Biology, 18(6): 1781–1796.
Erickson HP. 2009. Size and shape of protein molecules at the nanometer level determined by sedimentation, gel filtration, and electron microscopy. Biological Procedures Online, 11: 32–51.
Fadda E, and Woods RJ. 2010. Molecular simulations of carbohydrates and protein–carbohydrate interactions: motivation, issues and prospects. Drug Discovery Today, 15(15–16): 596–609.
Fenn J, and Raskino M. 2008. Mastering the hype cycle: how to choose the right innovation at the right time. Harvard Business Press, Boston, Massachusetts.
Fox CL, Juan J, and Albert RM. 1996. Phytolith analysis on dental calculus, enamel surface, and burial soil: information about diet and paleoenvironment. American Journal of Physical Anthropology, 101(1): 101–113.
Gallant DJ, Bouchet B, and Baldwin PM. 1997. Microscopy of starch: evidence of a new level of granule organization. Carbohydrate Polymers, 32: 177–191.
García-Díaz S, Hernández-Jaimes C, Escalona-Buendía H, Bello-Pérez LA, Vernon-Carter EJ, and Alvarex-Ramirez J. 2016. Effects of CaCO3 treatment on the morphology, crystallinity, rheology and hydrolysis of gelatinized maize starch dispersions. Food Chemistry, 207: 139–147.
García-Granero JJ, Lancelotti C, Madella M, Ajitprasad P, Crowther A, Korisettar R, et al. 2016. Millets and herders: the origins of plant cultivation in semiarid north Gujarat (India). Current Anthropology, 57(2): 149–173.
Golubic S, and Schneider J. 2003. Microbial endoliths as internal biofilms. In Fossil and recent biofilms: a natural history of life on Earth. Edited by WE Krumbein, DM Paterson, and GA Zavarzin Springer, Dordrecht, South Holland, the Netherlands. pp: 249–263.
Gough BM, and Pybus JN. 1973. Effect of metal cations on the swelling and gelatinization behaviour of large wheat starch granules. Starch/Stärke, 25(4): 123–130.
Gupta NA, and Briggs DEG. 2011. Taphonomy of animal organic skeletons through time. In Taphonomy: aims & scope topics in geobiology. Edited by PA Allison and DJ Bottjer. Springer, Dordrecht, South Holland, the Netherlands. pp. 199–221.
Gupta NS. 2015. Plant biopolymer–geopolymer: organic diagenesis and kerogen formation. Frontiers in Materials, 2: 61.
Gupta NS, and Pancost RD. 2004. Biomolecular and physical taphonomy of angiosperm leaf during early decay: implications for fossilization. PALAIOS, 19: 428–440.
Gupta NS, Michels R, Biggs DE, Collinson ME, Evershed RP, and Pancost RD. 2007. Experimental evidence for the formation of geomacromolecules from plant leaf lipids. Organic Geochemistry, 38(1): 28–36.
Gupta NS, Yang H, Leng Q, Briggs DE, Cody GD, and Summons RE. 2009. Diagenesis of plant biopolymers: decay and macromolecular preservation of Metasequoia. Organic Geochemistry, 40(7): 802–809.
Gupta R, Gigras P, Mohapatra H, Goswami VK, and Chauhan B. 2003. Microbial α-amylases: a biotechnological perspective. Process Biochemistry, 38(11): 1599–1616.
Hardy K, Blakeney T, Copeland L, Kirkham J, Wrangham R, and Collins M. 2009. Starch granules, dental calculus and new perspectives on ancient diet. Journal of Archaeological Science, 36(2): 248–255.
Hardy K, Buckley S, Collins M, Estalrrich A, Brothwell D, Copeland L, et al. 2012. Neanderthal medics? Evidence for food, cooking, and medicinal plants entrapped in dental calculus. Naturwissenschaften, 99(8): 617–626.
Hardy K, Radini A, Buckly S, Blasco R, Copeland L, Burjachs F, et al. 2016. Diet and environment 1.2 million years ago revealed through analysis of dental calculus from Europe’s oldest hominin at Sima del Elefante, Spain. Naturwissenschaften, 104(1–2): 2.
Hart TC. 2011. Evaluating the usefulness of phytoliths and starch grains found on survey artifacts. Journal of Archaeological Science, 38(12): 3244–3253.
Haslam M. 2004. The decomposition of starch grains in soils: implications for archaeological residue analyses. Journal of Archaeological Science, 31(12): 1715–1734.
Hayashizaki J, Ban S, Nakagaki H, Okumura A, Yoshii S, and Robinson C. 2008. Site specific mineral composition and microstructure of human supra-gingival dental calculus. Archives of Oral Biology, 53(2): 168–174.
Henry AG. 2015. Formation and taphonomic processes affecting starch granules. In Method and theory in paleoethnobotany. Edited by JM Marston and C Warriner. University Press of Colorado, Boulder, Colorado. pp. 35–50.
Henry AG, and Piperno DR. 2008. Using plant microfossils from dental calculus to recover human diet: a case study from Tell al-Raqā’i Syria. Journal of Archaeological Science, 35(7): 1943–1950.
Henry AG, Hudson HF, and Piperno DR. 2010. Changes in starch grain morphologies from cooking. Journal of Archaeological Science, 36(3): 915–922.
Henry AG, Brooks AS, and Piperno DR. 2011. Microfossils in calculus demonstrate consumption of plants and cooked foods in Neanderthal diets (Shanidar III, Iraq; Spy I and II, Belgium). Proceedings of the National Academy of Sciences of the USA, 108(2): 486–491.
Hill SA, MacNaughtan W, Farhat IA, Noel TR, Parker R, Ring SG, et al. 2005. The effect of thermal history on the Maillard reaction in a glassy matrix. Journal of Agricultural and Food Chemistry, 53(26): 10213–10218.
Hoover R. 2000. Acid-treated starches. Food Reviews International, 16(3): 369–392.
Huang J, Chen Z, Xu Y, Li H, Liu S, Yang D, et al. 2014. Comparison of waxy and normal potato starch remaining granules after chemical surface gelatinization: pasting behavior and surface morphology. Carbohydrate Polymers, 102: 1001–1007.
Hutschenreuther A, Watzke J, Schmidt S, Büdel T, and Henry AG. 2017. Archaeological implications of the digestion of starches by soil bacteria: interaction among starches leads to differential preservation. Journal of Archaeological Science: Reports, 15: 95–108.
Hwang IG, Kim HY, Woo KS, Lee J, and Jeong HS. 2011. Biological activities of Maillard reaction products (MRPs) in a sugar–amino acid model system. Food Chemistry, 126(1): 221–227.
Jacobs H, and Delcour JA. 1998. Hydrothermal modifications of granular starch, with retention of the granular structure: a review. Journal of Agricultural and Food Chemistry, 46(8): 2895–2905.
Jane J-L. 1993. Mechanism of starch gelatinization in neutral salt solutions. Starch/Stärke, 45(5): 161–166.
Jane J-L, Kasemsuwan T, Leas S, Zobel H, and Robyt J. 1994. Anthology of starch granule morphology by Scanning Electron Microscopy. Starch/Stärke, 46(4): 121–129.
Kaiser K, and Guggenberger G. 2000. The role of DOM sorption to mineral surfaces in the preservation of organic matter in soils. Organic Geochemistry, 31(7–8): 711–725.
Kayisu K, and Hood LF. 1979. Effects of dehydration and storage conditions on the pancreatic alpha amylase susceptibility of various starches. Journal of Food Science, 44(6): 1728–1731.
Kealhofer L, Torrence R, and Fullagar R. 1999. Integrating phytoliths within use-wear/residue studies of stone tools. Journal of Archaeological Science, 26(5): 527–546.
Kislenko VN. 2002. Polymer adsorption at solid surfaces. In Adsorption: theory, modeling, and analysis. Edited by J Tóth. Marcel Dekker, New York City, New York. pp. 743–800.
Larter SR, and Douglas AG. 1980. Melanoidins—kerogen precursors and geochemical lipid sinks: a study using pyrolysis gas chromatography (PGC). Geochimica et Cosmochimica Acta, 44(12): 2087–2095.
Laurence AR, Thoms AV, Bryant VM, and McDonough C. 2011. Airborne starch granules as a potential contamination source at archaeological sites. Journal of Ethnobiology, 31(2): 213–232.
Lee E-S, Kang S-M, Ko H-Y, Kwon H-K, and Kim B-I. 2013. Association between the cariogenicity of a dental microcosm biofilm and its red fluorescence detected by Quantitative Light-induced Fluorescence-Digital (QLF-D). Journal of Dentistry, 41(12): 1264–1270.
Lee-Thorp J. 2002. Two decades of progress towards understanding fossilization processes and isotopic signals in calcified tissue minerals. Archaeometry, 44(3): 435–446.
Limbach HJ, and Kremer K. 2006. Multi-scale modelling of polymers: perspectives for food materials. Trends in Food Science & Technology, 17(5): 215–219.
Locatelli ER. 2014. The exceptional preservation of plant fossils: a review of taphonomic pathways and biases in the fossil record. The Paleontological Society Papers, 20: 237–258.
Loy T, and Barton H. 2006. Post-excavation contamination and measures for prevention. In Ancient starch research. Edited by R Torrence and H Barton. Left Coast Press, Walnut Creek, California. pp. 165–167.
Loy T, Spriggs M, and Wickler S. 1992. Direct evidence for human use of plants 28,000 years ago: starch residues on stone artefacts from the northern Solomon Islands. Antiquity, 66(253): 898–912.
Luef B, Frischkorn KR, Wrighton KC, Holman H-YN, Birarda G, Thomas BC, et al. 2015. Diverse uncultivated ultra-small bacterial cells in groundwater. Nature Communications, 6: 6372.
Lützow MV, Kögel-Knabner I, Ekschmitt K, Matzner E, Guggenberger G, Marschner B, et al. 2006. Stabilization of organic matter in temperate soils: mechanisms and their relevance under different soil conditions—a review. European Journal of Soil Science, 57(4): 426–445.
McKnight RA, Shamay A, Sankaran L, Wall RJ, and Hennighausen L. 1992. Matrix-attachment regions can impart position-independent regulation of a tissue-specific gene in transgenic mice. Proceedings of the National Academy of Sciences of the USA, 89(15): 6943–6947.
Mehta D, and Satyanarayana T. 2016. Bacterial and archaeal α-amylases: diversity and amelioration of the desirable characteristics for industrial applications. Frontiers in Microbioly, 7: 1129.
Mercader J. 2009. Mozambican grass seed consumption during the Middle Stone Age. Science, 326(5960): 1680–1683.
Mercader J, Abtosway M, Baquedano E, Bird RW, Diez-Martin F, Domínguez-Rodrigo M, et al. 2017. Starch contamination landscapes in field archaeology: Olduvai Gorge, Tanzania. BOREAS, 46(4): 918–934.
Mercader J, Abtosway M, Bird RW, Bundala M, Clarke S, Favreau J, et al. 2018a. Morphometrics of starch granules from Sub-Saharan plants and the identification of ancient starch. Federated Research Data Repository.
Mercader J, Abtosway M, Bird RW, Bundala M, Clarke S, Inwood J, et al. 2018b. Morphometrics of starch granules from Sub-Saharan plants and the taxonomic identification of ancient starch. Open Science Framework preprint.
Meyer A. 1895. Untersuchangen Über die Stäkekorner. Fisher, Jena, Germany.
Molinero V, and Goddard WA. 2004. M3B: a coarse grain force field for molecular simulations of malto-oligosaccharides and their water mixtures. The Journal of Physical Chemistry B, 108(4): 1414–1427.
Nageli C, and Nageli KW. 1858. Die stärkekörner: morphologische, physiologische, chemisch-physicalische und systematisch-botanische. Nomographie, Zurich, Switzerland.
Oehler JH, and Schopf JW. 1971. Artificial microfossils: experimental studies of permineralization of blue-green algae in silica. Science, 174(4015): 1229–1231.
Olayinka OO, Adebowale KO, and Olu-Owolabi IB. 2013. Physicochemical properties, morphological and X-ray pattern of chemically modified white sorghum starch (Bicolor-Moench). Journal of Food Science and Technology, 50(1): 70–77.
Pacton M, Fiet N, and Gorin GE. 2007. Bacterial activity and preservation of sedimentary organic matter: the role of exopolymeric substances. Geomicrobiology Journal, 24(7–8): 571–581.
Pandey A, Nigam P, Soccol CR, Soccol VT, Singh D, and Mohan R. 2000. Advances in microbial amylases. Biotechnology and Applied Biochemistry, 31: 135–152.
Park I-M, Ibáñez AM, Zhong F, and Shoemaker CF. 2007. Gelatinization and pasting properties of waxy and non-waxy rice starches. Starch/Stärke, 59(8): 388–396.
Pearsall DM. 2015. Paleoethnobotany: a handbook of procedures. 3rd ed. Left Coast Press, Walnut Creek, California.
Pedergnana A, and Ollé A. 2017. Building an experimental comparative reference collection for lithic micro-residue analysis based on a multi-analytical approach. Journal of Archaeological Method and Theory, 25(1): 117–154.
Pedergnana A, Asryan L, Fernández-Marchena JL, and Ollé A. 2016. Modern contaminants affecting microscopic residue analysis on stone tools: a word of caution. Micron, 86: 1–21.
Pérez S, Baldwin PM, and Gallant DJ. 2009. Structural features of starch granules I. 3rd ed. In Starch. Edited by J BeMiller and Whistler. Academic Press, Elsevier, Amsterdam, The Netherlands. pp. 149–192.
Perry L, Sandweiss D, Piperno DR, Rademaker K, Malpass MA, Umire A, et al. 2006. Early maize agriculture and interzonal interaction in southern Peru. Nature, 440(7080): 76–79.
Perry L, Dickau R, Zarrillo S, Holst I, Pearsall DM, Piperno DR, et al. 2007. Starch fossils and the domestication and dispersal of chili peppers (Capsicum spp. L.) in the Americas. Science, 315(5814): 986–988.
Piperno DR, Ranere AJ, Holst I, and Hansell P. 2000. Starch grains reveal early root crop horticulture in the Panamanian tropical forest. Nature, 407(6806): 894–897.
Piperno DR, Weiss E, Holst I, and Nadel D. 2004. Processing of wild cereal grains in the Upper Palaeolithic revealed by starch grain analysis. Nature, 430(700): 670–673.
Piperno DR, Ranere AJ, Holst I, Iriarte J, and Dickau R. 2009. Starch grain and phytolith evidence for early ninth millennium B.P. maize from the Central Balsas river valley, Mexico. Proceedings of the National Academy of Sciences of the USA, 106(13): 5019–5024.
Power RC, Salazar-García DC, Wittig RM, and Henry AG. 2014. Assessing use and suitability of scanning electron microscopy in the analysis of micro remains in dental calculus. Journal of Archaeological Science, 49: 160–169.
Ratnayake WS, and Jackson DS. 2008. Chapter 5 Starch gelatinization. Advances in Food and Nutrition Research, 55: 221–268.
Reichert ET. 1913. The differentiation and specificity of starches in relation to genera, species, etc. Carnegie Institution of Washington, Washington, D.C.
Rots V, Hayes E, Cnuts D, Lepers C, and Fullagar R. 2016. Making sense of residues on flaked stone artefacts: learning from blind tests. PLoS ONE, 11(3): e0150437.
Salazar-García DC, Power RC, Serra AS, Villaverde V, Walker MJ, and Henry AG. 2013. Neanderthal diets in central and southeastern Mediterranean Iberia. Quaternary International, 318: 3–18.
Saranraj P, and Stella D. 2013. Fungal amylase—a review. International Journal of Microbiological Research, 4(2): 203–211.
Schmidt MW, Torn MS, Abiven S, Dittmar T, Guggenberger G, Janssens MK, et al. 2011. Persistence of soil organic matter as an ecosystem property. Nature, 478(7367): 49–56.
Schweitzer MH. 2011. Soft tissue preservation in terrestrial Mesozoic vertebrates. Annual Review of Earth and Planetary Sciences, 39: 187–216.
Singh J, Dartois A, and Kaur L. 2010. Starch digestibility in food matrix: a review. Trends in Food Science & Technology, 21: 168–180.
Smith SY, and Stockey RA. 2002. Permineralized pine cones from the Cretaceous of Vancouver Island, British Columbia. International Journal of Plant Sciences, 163(1): 185–196.
Tester RF, Qi X, and Karkalas J. 2006. Hydrolysis of native starches with amylases. Animal Feed Science and Technology, 130(1–2): 39–54.
Torrence R, and Barton H. 2006. Ancient starch research. Left Coast Press, Walnut Creek, California.
Townson JL, Lin YS, Chou SS, Awad YH, Coker EN, Brinker CJ, et al. 2014. Synthetic fossilization of soft biological tissues and their shape-preserving transformation into silica or electron-conductive replicas. Nature Communications, 5: 5665.
Ugent D, Pozorski S, and Pozorski T. 1982. Archaeological potato tuber remains from the Casma Valley of Peru. Economic Botany, 36(2): 182–192.
Wang S, and Copeland L. 2013. Molecular disassembly of starch granules during gelatinization and its effect on starch digestibility: a review. Food & Function, 4(11): 1564–1580.
Wang S, and Copeland L. 2015. Effect of acid hydrolysis on starch structure and functionality: a review. Critical Reviews in Food Science and Nutrition, 55(8): 1081–1097.
Wang W, Zhou H, Yang H, Zhao S, Liu Y, and Liu R. 2017. Effects of salts on the gelatinization and retrogradation properties of maize starch and waxy maize starch. Food Chemistry, 214: 319–327.
Wilkinson HP. 1983. Starch grain casts and moulds in Eocene (tertiary) fossil mangrove hypocotyls. Annals of Botany, 51: 39–45.
Wollast R, Mackenzie FT, and Bricker OP. 1968. Experimental precipitation and genesis of sepiolite at earth-surface conditions. The American Mineralogist, 53: 1645–1661.
Xhauflair H, Pawlik A, Forestier H, Saos T, Dizon E, and Gaillard C. 2017. Use-related or contamination? Residue and use-wear mapping on stone tools used for experimental processing of plants from Southeast Asia. Quaternary International, 427: 80–93.
Zhu XW, Gayin J, Chatel F, Dewettinck K, and Van der Meeren P. 2009. Influence of electrolytes on the heat-induced swelling of aqueous dispersions of native wheat starch granules. Food Hydrocolloids, 23(8): 2204–2211.
Information & Authors
Information
Published In
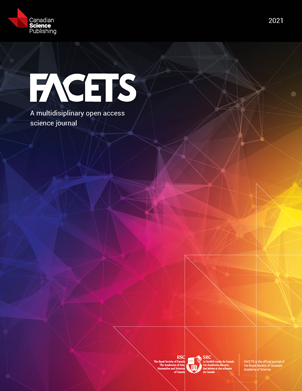
FACETS
Volume 3 • Number 1 • October 2018
Pages: 777 - 798
Editor: Tom A. Al
History
Received: 4 January 2018
Accepted: 25 May 2018
Version of record online: 30 July 2018
Copyright
© 2018 Mercader et al. This work is licensed under a Creative Commons Attribution 4.0 International License (CC BY 4.0), which permits unrestricted use, distribution, and reproduction in any medium, provided the original author(s) and source are credited.
Data Availability Statement
All relevant data are within the paper.
Key Words
Sections
Subjects
Plain Language Summary
Identifying starch in deep time: Designing a roadmap for archaeological starch research
Authors
Author Contributions
All conceived and designed the study.
All performed the experiments/collected the data.
All analyzed and interpreted the data.
JM, PD, SL, RS, and RT contributed resources.
All drafted or revised the manuscript.
Competing Interests
JM is currently serving as a Subject Editor for FACETS, but was not involved in review or editorial decisions regarding this manuscript.
Metrics & Citations
Metrics
Other Metrics
Citations
Cite As
Julio Mercader, Tolutope Akeju, Melisa Brown, Mariam Bundala, Matthew J. Collins, Les Copeland, Alison Crowther, Peter Dunfield, Amanda Henry, Jamie Inwood, Makarius Itambu, Joong-Jae Kim, Steve Larter, Laura Longo, Thomas Oldenburg, Robert Patalano, Ramaswami Sammynaiken, María Soto, Robert Tyler, and Hermine Xhauflair. 2018. Exaggerated expectations in ancient starch research and the need for new taphonomic and authenticity criteria. FACETS.
3(1): 777-798. https://doi.org/10.1139/facets-2017-0126
Export Citations
If you have the appropriate software installed, you can download article citation data to the citation manager of your choice. Simply select your manager software from the list below and click Download.
Cited by
1. A Multi-Dimensional Approach to Investigate Use-Related Biogenic Residues on Palaeolithic Ground Stone Tools
2. Earliest Evidence in the Philippines of Life Under the Canopy: Plant Technology and Use of Forest Resources by Our Species
3. Traceology
4. Archaeobotany: Microscopic and Molecular Techniques
5. Agricultural Fields
6. What about Apatite? Possibilities and Limitations of Recognising Bone Mineral Residues on Stone Tools
7. Chemical analysis of pottery reveals the transition from a maritime to a plant-based economy in pre-colonial coastal Brazil
8. Morpho-chemical characterization of individual ancient starches retrieved on ground stone tools from Palaeolithic sites in the Pontic steppe
9. Decodificando la memoria artefactual desde el sur de Sudamérica. Fortalezas del análisis integral de artefactos líticos
10. Archaeobotany
11. Editorial: Ancient starch remains and prehistoric human subsistence
12. Neolithic dental calculi provide evidence for environmental proxies and consumption of wild edible fruits and herbs in central Apennines
13. Morphometric Identification of Starch Granules From Archaeological Contexts: Diagnostic Characteristics of Seven Major North American Plant Families
14. Investigating Biases Associated With Dietary Starch Incorporation and Retention With an Oral Biofilm Model
15. Coupling the beams: How controlled extraction methods and FTIR-spectroscopy, OM and SEM reveal the grinding of starchy plants in the Pontic steppe 36,000 years ago
16. The use of plants by Neanderthals as food, medicine, and raw materials
17. Evidence from plant starch residues of the function of early pottery and the plant diet of Neolithic inhabitants of Inner Mongolia, North China
18. Diet and Lifestyle in the First Villages of the Middle Preceramic: Insights from Stable Isotope and Osteological Analyses of Human Remains from Paloma, Chilca I, La Yerba III, and Morro I
19. A novel route for identifying starch diagenetic products in the archaeological record
20. The challenge of the unique and unprecedented, comment on Agnihorti et al. (2021)
21. Developing a Reference Collection for Starch Grain Analysis in Early Neolithic Western Temperate Europe
22. Aurignacian grinding stone from Surein I (Crimea): “trace-ing” the roots of starch-based diet
23. Sandstone Ground Stone Technology: a Multi-level Use Wear and Residue Approach to Investigate the Function of Pounding and Grinding Tools
24. Food practices of the first farmers of Europe: Combined use-wear and microbotanical studies of Early Neolithic grinding tools from the Paris Basin
25. Effects of acetolysis on starch granules
26. Holocene grinding stones at Madjedbebe reveal the processing of starchy plant taxa and animal tissue
27. Effects of chemical pre-treatments on modified starch granules: Recommendations for dental calculus decalcification for ancient starch research
28. Starchy food residue on a potsherd from a late Holocene hunter-gatherer site in Argentine Patagonia: towards the visibility of wild underground storage organs
29. Microbotanical evidence for the spread of cereal use during the Mesolithic-Neolithic transition in the Southeastern Europe (Danube Gorges): Data from dental calculus analysis
30. Starch taphonomy, equifinality and the importance of context: Some notes on the identification of food processing through starch grain analysis
31. Using 3D digital microscopy and SEM-EDX for in-situ residue analysis: A multi-analytical contextual approach on experimental stone tools
32. Investigating Plant Micro-Remains Embedded in Dental Calculus of the Phoenician Inhabitants of Motya (Sicily, Italy)
33. “All that glitters is not gold”: Evaluating the Nature of the Relationship Between Archeological Residues and Stone Tool Function
34. Direct morpho-chemical characterization of elusive plant residues from Aurignacian Pontic Steppe ground stones
35. First direct evidence of wild plant grinding process from the Holocene Sahara: Use-wear and plant micro-residue analysis on ground stone tools from the Farafra Oasis, Egypt
36. Micrometrics and Morphological Properties of Starch
37. Influence of grinding on the preservation of starch grains from rice
38. Taphonomy of Plant Micro-remains in Environmental Archaeology
39. Starch Granules as Markers of Diet and Behavior
40. Archaeological Plant Remains
41. Taphonomy of Plant Micro-remains in Environmental Archaeology
42. History of Starch Research
43. The application of 3D modeling and spatial analysis in the study of groundstones used in wild plants processing
44. Structural characterization and decontamination of dental calculus for ancient starch research
45. Late Middle Stone Age Behavior and Environments at Chaminade I (Karonga, Malawi)
46. Micro-fossil analysis of Mesolithic human dental calculus, Motala, Sweden - Indications of health status and paleo-diet
47. Microscopic use-wear and residue analysis of stone reamers recovered from the early Holocene layer of the Eel Point site (CA-SCLI-43) on San Clemente Island, California
48. Oilseeds, spices, fruits and flavour in the Indus Civilisation
49. Morphometrics of Starch Granules From Sub-Saharan Plants and the Taxonomic Identification of Ancient Starch