Opportunities and trade-offs for expanding agriculture in Canada’s North: an ecosystem service perspective
Abstract
Climate change will create warmer temperatures, greater precipitation, and longer growing seasons in northern latitudes making agriculture increasingly possible in boreal regions. To assess the potential of any such expansion, this paper provides a first-order approximation of how much land could become suitable for four staple crops (corn, potato, soy, and wheat) in Canada by 2080. In addition, we estimate how the environmental trade-offs of northern agricultural expansion will impact critical ecosystem services. Primarily, we evaluate how the regulatory ecosystem services of carbon storage and sequestration and the habitat services supporting biodiversity would be traded for the provisioning services of food production. Here we show that under climate change projected by Canadian Earth System Model (CanESM2) Representative Concentration Pathway 4.5, ∼1.85 million km2 of land may become suitable for farming in Canada’s North, which, if utilized, would lead to the release of ∼15 gigatonnes of carbon if all forests and wetlands are cleared and plowed. These land-use changes would also have profound implications for Indigenous sovereignty and the governance of protected and conserved areas in Canada. These results highlight that research is urgently needed so that stakeholders can become aware of the scope of potential economic opportunities, cultural issues, and environmental trade-offs required for agricultural sustainability in Canada.
Introduction
Climate change creates opportunities for agricultural production in new regions, particularly those at higher latitudes and altitudes due to warmer temperatures and precipitation levels (Palomo 2017; King et al. 2018; Li et al 2018; Hannah et al. 2020a, 2020b). Globally, it is estimated that climate change will increase the amount of potentially suitable cropland by a total of 15.6 million km2 by 2100, predominantly in the Northern Hemisphere (Zabel et al. 2014). Hannah et al. (2020a) suggested that about 15 million km2 of our globe, about one-third of which will be located in Canada’s North, could become climatically viable for farmland by 2060–2080 as the growing season expands due to increasing temperature and rainfall. King et al. (2018) advised that by 2099, roughly 76% of boreal regions might reach appropriate growing degree days sufficient for small cereal crops, with a 1200 km northward latitudinal shift of the leading edge. Factors such as soil quality, terrain, infrastructure, and innovations in agricultural technology such as fast-maturing commodity crops that increase productivity in marginal regions will be major determinants of which of these frontiers will actually be cultivated (Power 2008; Council of Canadian Academies 2014; KC et al. 2020).
Relative to 1986–2005, by 2081–2100, average annual temperatures are projected to increase in Canada by 1.8 °C and 6.3 °C, under Representative Concentration Pathway (RCP) 2.6 and RCP 8.5, respectively, and increase in Northern Canada by 2.1 °C and 7.8 °C, under RCP 2.6 and RCP 8.5, respectively (Government of Canada 2020b). Similarly, relative to 1986–2005, by 2081–2100, normalized annual precipitation is projected to increase in Canada by 6.8% and 24.2%, under RCP 2.6 and RCP 8.5, respectively, and in Northern Canada by 9.4% and 33.3%, under RCP 2.6 and RCP 8.5, respectively (IPCC 2014; Government of Canada 2020b).
Emerging literature focuses on identifying and evaluating what are known as Climate Change Driven Agricultural Frontiers (CCDAFs)—defined as areas that are not currently cultivated or suitable for agriculture presently, but that may become suitable for agriculture in the future due to changing climatic patterns (Hannah et al. 2020a). It is unclear if CCDAFs will yield a net positive or negative impact on economic development and ecosystem services in Canada. Further research is needed to understand the impacts of agricultural frontier expansion across various dimensions, including food security, Indigenous sovereignty, ecosystem services, and economic development (see Ray et al. 2013). For instance, cultivating crops in CCDAFs could substantially expand global food production and potentially alleviate food insecurity in northern communities while contributing to much-needed economic development. For example, producing fresh, locally grown, nutrient-rich food could create jobs and help address the high rate of food insecurity in the Northwest Territories, Canada (NWT Bureau of Statistics 2014).
On the other hand, landscape transformation in Canada’s northern regions could also significantly impact many ecosystem services and affect Indigenous sovereignty. Ecosystem services are classified into four categories: provisioning services (e.g., producing/extracting food, fibre, and fuel resources), regulating services (that support stability in natural systems and mitigate extreme events), habitat or supportive services (that facilitate other ecosystem services by maintaining habitat structure and biodiversity), and cultural services (including the often intangible benefits to humans when people interact physically, emotionally, or spiritually with nature). Additionally, the prospect of widespread cultivation across much of today’s boreal forest raises concerns about whether Indigenous land claims and rights of self-determination will be adequately acknowledged and respected by the federal and provincial governments or the private sector.
Realizing the potential benefits of CCDAFs for provisioning service will require carefully exploring trade-offs between existing regulating, supportive, and cultural ecosystem services such as climate regulation, water resource maintenance, biodiversity support, and health and spirituality support (Turner et al. 2003; Palomo 2017; Hannah et al. 2020a). For instance, land-use changes that increase provisioning ecosystem services may result in a loss of biodiversity and increased greenhouse gas emissions from newly ploughed carbon-rich land (Lawrence et al. 2015; Natali et al. 2015). New research suggests that a complete global expansion into CCDAFs could generate enough carbon emissions to preclude limiting climate change to 1.5 °C of warming, threaten water resources for billions of people, and severely degrade ecosystems that support biodiversity hotspots (Molotoks et al. 2017; Hannah et al. 2020a). Moreover, the impacts of converting land to agriculture on hydrological drainage patterns could make ecosystems such as peatlands more vulnerable to drying and wildfires (Turetsky et al. 2015). There may also be impacts to ecosystem services that are especially culturally and economically significant for First Nations, Métis, and Inuit peoples of Canada’s northern areas. Consequently, managing trade-offs between expanding food production northward and the degradation or destruction of these ecosystem services will be a significant dilemma for Indigenous, federal, provincial, and regional policymakers.
Our research maps ecosystem services to provide insight into how land transformation could potentially disrupt existing ecological processes across the Canadian North. Using ecosystem services as a conceptual model through which to examine agricultural frontiers offers several advantages. First, it allows us to compare seemingly disparate aspects of how ecosystems impact human systems—such as food or fuel production and spiritual services—within one framework. Second, it allows us to frame biophysical processes within the broader context of socioeconomic changes and environmental governance. Climate change impacts intersect with economic development, environmental change, and Indigenous–Crown governance relationships. So, the ability to study the effects of climate change on these agricultural frontiers benefits greatly from the cross-disciplinary framework provided by ecosystem services.
In this paper, we conduct a first-order approximation of CCDAFs’ spatial limits within Canada using multiple climate change scenarios. We then examine what ecosystem service trade-offs would be impacted by expanding conventional agricultural production into CCDAFs in Canada, and how those trade-offs could create cascading impacts through human and nonhuman environments. Given this context, this paper seeks to answer the following questions. (i) What will be the extent of agricultural frontiers in Canada by 2080? (ii) What ecosystem service trade-offs would result from the utilization of identified agricultural frontiers?
In answering these questions, this paper contributes to a growing body of literature using models to identify potential areas suitable for agricultural activities due to climate change (Bootsma 2013; Zabel et al. 2014; Molotoks et al. 2017; King et al. 2018). More specifically, this paper estimates the potential impact on ecosystem services if these areas were to be used for four key commodity crops: potatoes, wheat, corn, and soy. We build on current literature by: (i) using high-resolution climate models to identify the potential areas suitable for expanding these four commodities in Northern Canada; (ii) using land use, soil carbon, and land cover data to conduct a preliminary evaluation of some potential ecosystem trade-offs in the northern region of Canada; (iii) reflecting on research gaps that need to be filled to make more precise estimations on potential agricultural areas and their other probable ecosystem trade-offs; and (iv) highlighting the significance of this issue for First Nations, Métis, and Inuit rights and sovereignty.
Data and Methods
Data
We identify potential agricultural frontiers within Canada by using three overarching biophysical variables: climate, soil, and terrain (slope, elevation), and two anthropogenic variables: distribution of agricultural land cover by crop and existing road infrastructure. A summary of the data is presented in Supplementary Material 1, Table S1.
Biophysical variables
To determine present-day crop suitability, climate data for the year 2018 was retrieved from Natural Resources Canada (NRC) upon request (Government of Canada 2020b). Climate data for a single year was used instead of a multi-decade average to ensure that the “present-day” climate matched the climate of the 2018 Canada Annual Crop Inventory. NRC uses “thin plate smoothing splines” using the ANUSPLIN climate modelling software to produce their annual grids at a resolution of 60 arc-seconds (McKenney et al. 2011; Government of Canada 2020b). These climate grids are spatially continuous and are commonly applied in agriculture and forestry (McKenney et al. 2011; Government of Canada 2020b). NRC climate grids were downscaled to 30 arc-seconds—roughly 1 km2—using “bilinear interpolation” resampling (Hijmans 2020). The 2018 climate variables include:
•
total precipitation during the growing season of 2018 (millimeters),
•
the number of days of the growing season in 20181, and
•
the mean temperature for growing season during 2018 (°C).
A 30-year projected climate data set (2071–2100) was also retrieved from NRC upon request (Government of Canada 2020b), which was averaged to 2080. The projected climate variables include total precipitation throughout the growing season of 2071–2100 in millimeters, the number of days of the growing season in 2071–2100, and mean temperature for the growing seasons throughout 2071–2100 in °C (Government of Canada 2020b). Each of these variables was collected as an average of annual projections for the 30-year range. The global climate model that NRC used to generate these averaged projected climate grids was based on the Canadian Earth System Model (CanESM2) model, representing the RCP for the 4.5 scenarios (Government of Canada 2020b). The resolution of the projected climate data was in 300 arc-seconds and was downscaled to 30 arc-seconds—roughly 1 km2—using “bilinear interpolation” resampling (Hijmans 2020). Hereon, this data will be referred to as the 2080 projected climate model.
Soil data were retrieved from the Food and Agriculture Organization of the United Nations (FAO), using the FAO’s Soil Portal (FAO 2009). Within the Soil Portal, the 30 arc-second Harmonized World Soil Database V 1.2 was used and linked to harmonized soil property data (FAO 2009). Topsoil (0–30 cm) physical and chemical properties used in this study include gravel content, United States Department of Agriculture texture classification, organic carbon, pH, cation exchange capacity, base saturation, calcium carbonate, gypsum, sodicity, salinity, and drainage class (FAO 2009). These soil parameters were used to conduct a land suitability assessment of the four major crops for 2018 and for the 2080 projected climate model.
Elevation and slope were used as topographic variables. Elevation data were retrieved from WorldClim Global Climate Data in a 30 arc-second raster (Fick and Hijmans 2017). A built-in algorithm proposed by Wang and Liu (2006) was used in SAGA GIS software to ensure no surface depressions were present in the elevation data (Wang and Liu 2006; Conrad et al. 2015). After creating this “depression-less” elevation data, slopes were then derived using the SAGA GIS software (Conrad et al. 2015). The elevation and slope data were also used to do a land suitability assessment of the four major crops during the year 2018 and for the 2080 projected climate model.
Anthropogenic variables
Existing road infrastructure was defined according to Statistics Canada (2020), an intercensal database that digitally represents Canada’s road network.
The distribution of agricultural land cover by crop was determined through the Canada Annual Crop Inventory, an annual assessment produced by Agriculture and Agri-Food Canada that maps the spatial extent of crop types in Canada. The Annual Crop Inventory utilizes a combination of RADARSAT-2 radar imagery and Landsat-8, Sentinel-2, and Gaofen-1 optical imagery to produce a 30-m resolution inventory with an overall target accuracy of 85% (Agriculture and Agri-Food Canada (AAFC) [dataset] 2018). Satellite data categorization is trained and validated primarily through annual Canadian crop insurance data with additional verification through ground-truth information. At the time of data collection, 2018 was the most recent available complete data set and was used for analysis.
1
NRC defines a growing season as beginning when the temperature is greater than or equal to 5°C for five consecutive days (after March 1) and ending when the average minimum temperature is less than −2°C (after August 1) (Government of Canada 2020b).
Methods
A five-step method was used to identify CCDAFs and their potential ecosystem service impacts. The process is outlined in Supplementary Material 2, Fig. S1. Briefly, Step 1 identified how climate change would affect the distribution of land suitable for the cultivation of corn, potatoes, soy, and wheat by 2080 in Canada. These crops were chosen because they represent four globally significant staple crops capable of being successfully cultivated at a commercial scale in Canada. Step 2 validated the models produced in Step 1 against the 2018 Canada Crop Inventory (Agriculture and Agri-Food Canada 2018 ; Supplementary Material 2, Fig. S2). Step 3 identified a 10-km buffer zone surrounding Canada’s road network (Supplementary Material 2, Fig. S3) to provide a limited suitability scenario based on the assumption that areas with access to the transportation infrastructure will most likely be developed. Importantly, since Canada’s road network will undoubtedly become more extensive by 2080, Step 3 creates a conservative scenario of emerging land suitability cultivating the four study crops based on development constraints imposed by infrastructure limitations. We propose a 10-km buffer of Canadian road infrastructures somewhat arbitrarily since there was no consensus in the literature about what an “ideal road proximity” should be for this scenario. Next, Step 4 used ESRI’s ArcGIS to calculate the geography of CCDAFs and current agricultural frontiers using both Model One and Model Two. Finally, Step 5 calculated the potential impact of developing all CCDAFs and just the areas within 10 km of a road in terms of two ecosystem services: loss of regulating carbon storage and sequestration services and impact on supportive or habitat services measured by leading tree species area, and two environmental governance factors: impact to protected and conserved areas andimpact within federally recognized Indigenous territories. We provide a more detailed explanation of our method below.
Step 1: Crop suitability analysis
Development of Model One
Model One was developed with a GIS-based weighted overlay technique (Kaliraj et al. 2015; Basharat et al. 2016; Awawdeh et al. 2018; Al-Anbari et al. 2018; Vázquez-Quintero et al. 2019; Tashayo et al. 2020) that assessed land suitability for the cultivation of corn, potatoes, soy, and wheat in Canada based on three variables: climate, soil, and topography. Using various sources (Sys et al. 1993, Tashayo et al. 2020, FAO 2021a, 2021b, New Brunswick Canada 2021; Ontario Ministry of Agriculture, Food and Rural Affairs 2021), a list of criteria consisting of 16 factors was developed (presented in Supplementary Material 1, Table S2) that specifies the conditions required for growing each of the four study crops. Each factor was assigned a score between 1 and 4 based on our identified suitability ranges, and each crop was assessed independently, producing a total of 64 scored suitability grids. A score of 1 represents land unsuitable for agriculture, a score of 2 represents marginally suitable land, a score of 3 represents moderately suitable land, and a score of 4 represents areas highly suitable for each crop.
Next, the scored suitability grids were weighted using a subjective approach (Vázquez-Quintero et al. 2019; Tashayo et al. 2020; Awawdeh et al. 2018; Basharat et al. 2016). Soil and topography factors were assigned 40% weight, and climate factors were assigned 60% weight in the model. Climate factors were assigned a greater weight since climatic conditions may preclude regions from supporting crop cultivation regardless of soil and topographic conditions. Finally, the weighted suitability scores were reclassified into a binary suitability grid; “moderately suitable” and “highly suitable” areas were classified as suitable for crop cultivation, whereas “not suitable” and “marginally suitable” areas were excluded from the final model.
Two sub-models were created using this method, which individually assessed the current suitability based on 2018 data and the 2080 projected climate model. Importantly, soil and topography data were kept consistent between the two sub-models, based on the assumption that these characteristics will not change significantly between now and 2080. For the current modelled suitability, 2018 climate data was used. For the projected crop suitability, average projected climate grids were created based on the CanESM2 model, representing the RCP for the 4.5 scenarios (Government of Canada 2020b). The modelled crop suitability for 2018 and the 2080 projected climate model are presented in Supplementary Material 2, Figs. S4–S6.
Development of Model Two
Model Two was produced using the 2018 Canada Crop Inventory (Agriculture and Agri-Food Canada 2018). This 30-m resolution data set that identifies the locations of all crops grown in Canada was generated by the Earth Observation Team of the Science and Technology Branch of Agriculture and Agri-food Canada using satellite imagery and decision-tree methodology and is validated using ground truth information (Agriculture and Agri-Food Canada 2018). The 2018 Canada Crop Inventory data set was further processed to ensure that the coordinate reference system and resolution matched the suitability input grids using “nearest neighbor” resampling (Hijmans 2020). The four study crops were extracted and overlaid with data from the 16-factor suitability grids. Histograms for each factor and each crop were produced to assess the explanatory power of the 16 factors on present-day crop distributions. Observing the frequencies in the histograms, we concluded that total precipitation, mean temperature, number of growing days during the growing season of 2018, and elevation hold the greatest explanatory power (Supplementary Material 1, Table S3). The four dominant factors were then used to conduct a second suitability analysis using Boolean analysis (Kaliraj et al. 2015) to identify suitable areas across all four dominant factor suitability grids (Table S3). Similarly, using the same criteria presented in Table S3, projected suitability grids were generated for each crop using the 2080 projected climate model and the 2018 soil and elevation data, and converted into binary suitability grids using the same method described above.
Step 2: Comparing modelled crop suitability with observed crop growing areas in 2018
The 2018 Canada Crop Inventory (Agriculture and Agri-Food Canada 2018) was compared against Model One and Model Two to validate both models’ accuracy. By overlaying the 2018 crop inventory data set with the land suitability maps made in Step 1, contingency tables were made to calculate the overall accuracy of the produced final binary suitability grids. Model One was found to be approximately 65%, 48%, 60%, and 68% accurate for corn, potato, soy, and wheat, respectively. Model Two was found to be approximately 95%, 92%, 94%, and 80% accurate for corn, potato, soy, and wheat, respectively. Boolean conditions reflecting the Canada Crop Inventory (Agriculture and Agri-Food Canada 2018) within Model Two explain the much higher accuracy than Model One.
However, Model Two underrepresents the true extent of crop suitability for each of our study crops because it assumes that all land suitable for each of these crops is being utilized for their cultivation. By developing a suitability model based on where crops are located for a single year, we fail to account for the plethora of factors that might preclude a crop from being cultivated in a given location, such as use for other crop cultivation or an alternative land use. Therefore, we present Model One and Model Two as counterparts representing an upper- and lower-bound estimate of CCDAFs.
Step 3: Estimating the geographic extent of agricultural frontiers
Using the modelled binary suitability grids for 2018 and the projected binary suitability grids for 2080 produced and validated in Steps 1 and 2, we estimated the geographic extent of two types of agricultural frontiers for each of the four study crops within ESRI’s ArcGIS. First and most importantly for this paper, CCDAFs were calculated using Boolean grid algebra as any area not currently utilized for any agricultural activity per the 2018 Canada Crop Inventory, is unsuitable for crop cultivation in 2018 per the modelled 2018 binary suitability grids and is projected to become suitable for crop cultivation in 2080 per the projected 2080 binary suitability grid. Secondly, current agricultural frontiers were identified as any areas suitable for crop cultivation in 2018 per the modelled 2018 binary suitability grids, but not currently utilized for any agricultural activity per the 2018 Canada Crop Inventory (Supplementary Material 2, Fig. S2). Pure CCDAFs were identified only in areas that contained at least one crop-specific CCDAF but not any current agricultural frontiers. We calculate all remaining results based on the spatial extent of pure CCDAFs. All agricultural frontier grids were scaled to a 30-arc second resolution using “nearest-neighbor” resampling (Hijmans 2020).
Fig 1.

Step 4: Identifying areas close to the transportation infrastructure
To capture the fact that areas with a better-developed transportation infrastructure are more likely to be developed by farmers, we calculated a second scenario for each of our models and sub-models, which restrict agricultural frontiers to areas within a 10-km radius of all 2016 Canadian road infrastructure (Statistics Canada 2020; Supplementary Material 2, Fig. S3) using Boolean raster algebra. The resolution and the coordinate reference system were adjusted to match the crop suitability grids produced in Steps 1 and 2 using nearest neighbor resampling (Hijmans 2020). Given that the road network in 2080 will likely be different from today, for the rest of this paper, we present results both within this 10 km radius of the contemporary road network—a lower-bound estimate—and for all CCDAFs—an upper-band estimate.
Step 5: Estimating environmental and social impacts
In the last step, we mapped and quantified the potential impact of the 2080 frontiers for four key impact indicators.
Carbon storage and sequestration
By clearing and tilling land, utilizing CCDAFs eliminates the existing carbon storage and sequestration process and releases stored soil carbon, which has profound implications for climate change mitigation (Lawrence et al. 2015; Natali et al. 2015). So, we estimated the amount of soil carbon stored within the top 30 cm of soil throughout all identified pure CCDAFs. Using gridded global data (Hengl et al. 2012; Supplementary Material 2, Fig. S7), we extracted soil data for all cells within the pure CCDAFs identified in Step 4. The total tonnage of soil carbon was then summed for each model and sub-model, both with and without road proximity restrictions. This estimates the range of possible carbon emissions that might result if all pure CCDAFs are cultivated.
Biodiversity maintenance
Maintaining intact and unfragmented habitats is essential for preserving biodiversity hotspots and mitigating the risk of wildfires and drought (Turetsky et al. 2015; Molotoks et al. 2017; Hannah et al. 2020a). To estimate the potential impact of utilizing pure CCDAFs on biodiversity and habitat diversity, we utilized key leading species data (Natural Resources Canada 2020; Supplementary Material 2, Fig. S8) to determine what habitats are proportionally at risk within pure CCDAFs.
Impacts within federally protected and conserved areas
Federally conserved and protected areas will likely limit the extent to which CCDAFs could be utilized. Therefore, we calculated the total extent of federally conserved and protected areas that intersect with pure CCDAFs by overlaying the outputs of Step 4 with all Canadian Protected and Conserved Areas (Government of Canada 2021). Total areas were then quantified for each model and sub-model, both with and without road proximity limits were then summed.
Impacts to federally recognized Indigenous territory
Indigenous land areas that intersect with potential new cropland were estimated by overlaying the existing federally recognized Indigenous territories within Canada (Government of Canada 2020a) with all pure CCDAFs identified (Step 4). Total areas intersecting both models and sub-models, both with and without road proximity limits, were summed.
Methodological limitations
We identify several significant limitations to our first-order approximation. First, data resolutions for several factors, most notably soil data, required scaling our results to a coarse resolution and effectively limiting the accuracy of our analysis. Second, the design of our method is partly subjective, mainly the weights assigned to our suitability factors. An alternative approach that could have been utilized to determine factor weights is an analytical hierarchy process (AHP) (Tashayo et al. 2020; Basharat et al. 2016; Al-Anbari et al. 2018), as demonstrated by Tashayo et al. (2020). Expanding this study using AHP presents an opportunity for future research. Delphi panel methodology is also worth considering and would help provide additional legitimacy to subjective decision-making during study design.
Results
Estimates of the geographic extent of agricultural frontiers
Overall, our results show that large areas of Canada become suitable for agriculture under the climate change presented by the CanESM2 climate model. Model One, which uses all 16 factors, suggests that CCDAFs will emerge much further morth than those that emerge in the four-factor Model Two. Nevertheless, both models show that large areas of the boreal forest will become suitable for farming by the end of the 21st century (Fig. 1). Under Model One, approximately 1.85 million km2 will become suitable for agriculture by 2080 in Canada. Approximately 152 000 km2 is found within 10 km of the current road network (Fig. 1, Table 1). The vast majority of this area is found in an east–west belt that extends across the three Canadian territories, Northern Québec, and Labrador. A second north–south belt of suitable CCDAFs emerge through the Rocky Mountains in British Columbia and the Yukon. Only a tiny proportion of CCDAFs identified by Model One fall within the other Canadian provinces (Fig. 1).
Table 1.
Model One (16 suitability criteria) | Model Two (4 suitability criteria) | |||
---|---|---|---|---|
Potential impacts | Total potential impact (% of total)a | Total potential impact within 10 km of major roads (% of total)b | Total potential impact (% of total)c | Total potential impact within 10 km of major roads (% of total)d |
Area of land that may become suitable for cropping by 2080 (km2) | 1 850 055 | 152 328 | 1 850 503 | 731 849 |
Gt of carbon stored in top 30 cm of soil for potential new cropland | 30.44 | 4.44 | 28.26 | 9.26 |
Area of land currently occupied by key tree species that intersect with potential new cropland (km2).e | ||||
Alpine Fir (Abies lasiocarpa) | 135 484 (5.6) | 28 061 (1.2) | 7 731 (0.3) | 4 932 (0.2) |
Balsam Fir (Abies balsamea) | 143 (<0.1) | 28 (<0.1) | 29 355 (1.8) | 23 375 (1.4) |
Black Spruce (Picea mariana) | 584 946 (15.3) | 18 524 (0.5) | 1 042 979 (27.2) | 217 291 (5.7) |
Douglas Fir (Pseudotsuga menziesii) | 2 630 (0.2) | 2 348 (0.2) | 19 156 (1.8) | 18 876 (1.8) |
Jack Pine (Pinus banksiana) | 21 461 (0.6) | 332 (<0.1) | 140 134 (3.9) | 19 516 (0.5) |
Lodgepole Pine (Pinus contorta) | 40 818 (1.3) | 21 167 (0.7) | 80 915 (2.7) | 55 903 (1.8) |
Tamarack (Larix laricina) | 13 18 (1.9) | 122 (0.2) | 45 077 (65.1) | 4 691 (6.8) |
Trembling Aspen (Populus tremuloides) | 15 547 (0.2) | 2 742 (<0.1) | 153 113 (1.7) | 76 589 (0.9) |
White Birch (Betula papyrifera) | 639 (0.7) | 30 (<0.1) | 26 063 (29.0) | 9 327 (10.4) |
White Spruce (Picea glauca) | 85 243 (2.9) | 20 439 (0.7) | 86 997 (3.0) | 31 695 (1.1) |
Protected and conservation areas that intersect with potential new cropland (km2) | 334 131 | 27 679 | 167 673 | 27 960 |
Indigenous Treaties lands that intersects with potential new cropland (km2) | 125 869 | 11 424 | 65 688 | 19 584 |
In terms of crop-specific CCDAFs, we observe that 1.08 million km2 for will emerge for corn cultivation (88 000 km2 within 10 km of a road), 1.75 million km2 for potato cultivation (147 000 km2 within 10 km of the road network), 1.23 million km2 for soy (82 000 km2 within 10 km of a road), and 504 000 km2 for wheat (55 200 km2 within 10 km of a road). Crop-specific geographic distribution is relatively uniform across the four crops with two exceptions. First, the range of potato’s CCDAF extends much further into Northern Québec than the other three crops. Second, wheat shows a much more limited extent than the other three crops, with hardly any distribution within the Northwest Territories and Nunavut.
Surprisingly, Model Two suggests like Model One, approximately 1.85 million km2 will become suitable for agriculture by 2080, contradicting our expectation that Model Two would provide a lower-bound estimate and Model One an upper-bound estimate (Table 1). Although the extent of CCDAFs is the same between each model, we find substantially geographic distributions. The distribution of CCDAFs within Model Two is within a singular belt that extends from northwestern Canada in the Northwest Territories through central Canada, northern Ontario, and central Québec. A small proportion of Model Two’s identified CCDAFs appear within the Rocky Mountains, and very little of the CCDAFs identified in the Yukon in Model One remain in Model Two. Additionally, a small proportion of CCDAFs extend into the Maritimes, including Nova Scotia and Labrador.
Model Two’s crop-specific results also differ considerably from Model One. Specifically, we observe that 1.09 million km2 for will emerge for corn cultivation (255 000 km2 within 10 km of a road), 1.56 million km2 for potato cultivation (415 000 km2 within 10 km of the road network), 503 000 km2 for soy (82 000 km2 within 10 km of a road), and 1.56 million km2 for wheat (447 000 km2 within 10 km of a road). The greatest crop-specific differences between Models One and Two are shifts in soy and wheat suitability, which essentially invert their total extent within projected CCDAFs under each model. Conversely, the extent of corn and potato remain roughly equivalent between the two models. We also find that Model Two shows a much greater extent of CCDAFs near roads, 580 000 km2 more than Model One. We attribute this observation to the latitudinal differences between the locations of CCDAFs of each model, which align with latitudinal differences between Canada’s road network density since Canada has a much denser road network in the south than in the north.
Potential impact of agricultural frontiers
In this section, we turn our attention to some of the potential implications of utilizing CCDAFs for agriculture on ecosystem services and potential governance conflicts. We focus on two specific ecosystem service impacts and two governance impacts directly related to ecosystem services. This is not intended to be an exhaustive or comprehensive treatment of potential impacts, in fact, our analysis is extremely limited in the broader context of recognized ecosystem services. Still, rather than providing a comprehensive analysis, we intend to provide a starting place for policy-relevant discussions on the nature of possible trade-offs between food security and sustainability.
Carbon storage and sequestration
Following a range of authors (e.g., see: Tilman 1999; Parry et al. 2004; Phelps et al. 2013; Laurance et al. 2014; Watson 2014; Popp et al. 2017), results presented in Table 1 show that one of the most significant consequences of developing new cropland in Northern Canada will be the implications of land cover conversions on carbon storage and sequestration. The models presented here suggest that as much as 15 GtC (Table 1 and Fig. 2) is stored in the top 30 cm of soils within identified CCDAFs, of which 1–4 GtC is within 10 km of a road. By contrast, Canada’s annual carbon emissions today are estimated to be ∼0.73 GtC annually (Environment and Climate Change Canada 2021). As such, one implication of the results presented in this paper is to highlight how developing these frontiers could represent major new sources of emissions for Canada, threatening global climate security and Canada’s international commitments to reducing national carbon emissions.
Fig 2.

Biodiversity maintenance
If all identified CCDAFs in Model One are converted to agricultural lands, our results show that 903 000 km2 of forest habitat would be cleared or degraded (104 000 km2 near roads). Several leading tree species would be heavily impacted by such development, mainly Black Spruce (Picea mariana) and Alpine Fir (Abies lasiocarpa). Model Two shows an even greater impact, with 1.7 km2 of forest habitat intersecting identified CCDAFs. Both Tamarack (Larix laricina) and White Birch (Betula papyrifera) would have large proportions of their habitat destroyed or degraded in this scenario. Destroying or degrading habitats with these leading species would reduce the capacity of boreal forest and other forest ecosystems to support biodiversity, maintain a regular hydrological cycle, and mitigate forest fire risks (Table 1 and Fig. 3). Quantifying the extent of these impacts is beyond the scope of this power.
Fig 3.

Impacts within federally protected and conserved areas
Currently, there are approximately 334 000 km2 identified as belonging to a federally protected or conserved area intersecting emerging CCDAFs, of which approximately 28 000 km2 lies within 10 km of a major road (Table 1 and Fig. 4). Therefore, this land may be considered ideally situated for rezoning and redevelopment as the climate warms.
Fig 4.

Impacts to federally recognized indigenous territory
Canada is made up of a large patchwork of unceded Indigenous territory or territory identified in treaties with Indigenous Nations. Our results show that up to 126 000 km2 or 10% of federally recognized Indigenous territory intersects identified CCDAFs of the lands that may become suitable for farming by 2080 fall within existing treaty territories (Table 1 and Fig. 5). Most of this territory is found within the Northwest Territories and Nunavut, indicating that CCDAFs could have substantial implications for northern food security and economic opportunities for Indigenous Nations.
Fig 5.

Discussion
Using agricultural, climate, soil, and topographic data to assess the geographic extent of emerging CCDAFs, this paper illustrates the magnitude the opportunity to expand agriculture northward and upslope in Canada and the potentially severe implications to ecosystem services and Indigenous sovereignty. We integrated several approaches to estimate and evaluate CCDAFs, but, despite differences between our approaches, the results of our research remain the same and suggest three findings. First, climate change will create vast expanses of land where agriculture could expand further North in Canada. Up to 1.85 million km2 could become newly viable for cultivation. Second, capitalizing on the opportunity to utilize CCDAFs would have potentially detrimental impacts on ecosystem services and environmental sustainability. Although likely unrealistic, converting the full extent of CCDAFs to agriculture would release up to 15 GtC from soil stored carbon and affect up to 1.66 million km2 of forest habitat. Third, the development of CCDAFs is founded on the need for the integration of Free, Prior, and Informed Consent per the United Nations Declaration on the Rights of Indigenous Peoples (UNDRIP) (United Nations General Assembly 2008) and adequate acknowledgement of Indigenous rights under section 35 of the Canadian constitution in all planning and decision-making on this issue. Notably, this issue is relevant becasue of the high proportion of federally recognized Indigenous territory that intersects identified CCDAFs.
While it may seem far-fetched to imagine a future where there is extensive soy, wheat, corn, or potato production in the far north, recent history suggests that such land-use transitions are not only possible but have also recently occurred, to the detriment of both Indigenous Communities as well as the environment. For example, until a few years ago, land that was thought not useable for agriculture—such as South America’s Amazon or southeast Asian peat soils—has been rapidly converted to agriculture thanks to a range of factors including weather, economic drivers, policies, and technology (Hannah et al. 2020a). The research presented in this paper suggests that similar scenarios could also happen in Canada’s far- and near-north. As such, this discussion proceeds by developing two key points. First, we reflect on the ways—or causal mechanisms—in which agricultural expansion can drive adverse outcomes for the environment and Indigenous communities. Second, we build on this discussion by exploring some of the implications of this current study to launch future research and policy discussion.
Exploring how agricultural expansion drives negative consequences
According to the literature, one of the most significant environmental impacts of expanding farmland is the release of below- and aboveground carbon and likely a reduction in carbon storage as new areas become climatically suitable for crops. In terms of ecosystem services, carbon storage is considered a regulatory service with preliminary estimates indicating ∼40.90 Gt of carbon resides in the top 30 cm of soil under potential agricultural frontiers. Owing to their immense carbon reserves, soil-bound carbon release in high latitudes is already a major concern (Crowther et al. 2016). The literature suggests that carbon is released quickly into the atmosphere following tillage, with estimates suggesting 25%–40% of total soil carbon can be released within five years of plowing (Davidson and Ackerman 1993). Other studies show that on average, ∼30% of soil carbon is lost when boreal forest ecosystems are converted and tilled; this would correspond with a loss of approximately 0.02 kg C/m2/year in northern agricultural systems (Bysouth 2020, personal communication), contrasting with the –0.01 to 0.09 kg C/m2/year accumulation rate reported for soils systems in forests across the western boreal biome (Walker et al. 2020). Therefore, our first order approximation estimation is that up to 15 GtC could be lost from Canada’s northern ecosystems. Walker et al. (2020) confirmed that this decomposition is likely to occur in the first 10 years following the conversion of forests to agriculture. This loss is primarily attributed to enhanced decomposition and soil erosion following active tilling and the removal of overstory vegetation that shade soils and provide structural stability (Lal 2002).
Expanding agricultural activity into CCDAFs would have serious implications for forest habitat integrity and tree species diversity. We found that between 903 000 km2 (Model One) and 1.66 million km2 (Model Two) intersect forest habitats, representing 49% and 90% of all identified CCDAFs, respectively. While these land-use types are likely the most amenable for conversion to farming in terms of soil, they also provide the richest wildlife habitat. Some species face greater proportional risks of habitat destruction than others, especially if Model Two proves to be a more accurate projection of CCDAFs. For example, in Model One, 15% of Black Spruce’s total range falls within CCDAFs; in Model Two, this percentage increases to 28%. Model Two also identifies that 65% of Tamarack-dominated habitats and 29% of White Birch-dominated habitats fall within projected CCDAFs. Consequently, complete utilization of CCDAFs would severely destroy, degrade, or fragment forest habitats vital for supporting local and regional biodiversity. The loss of such habitat services that maintain biodiversity and healthy ecosystems may have cascading effects on northern food security, as essential country foods, like caribou, are dependent on vast expanses of pristine rangelands (Festa-Bianchet et al. 2011). The loss of critical habitat that supports culturally significant foods and biodiversity itself would have to be weighed against increased local food security.
Finally, in terms of Indigenous sovereignty, we note that one of the key legacies of colonialism is the chronic failure of settler organizations, including researchers (such as the authors of this paper), industry, and the Crown, to fulsomely engage with the leaders of Indigenous Communities whose interests are often ignored and suppressed in planning and development processes (see chapter 1 in Mosby et al. 2020). As a result, and also in response to the Calls to Action from the Truth and Reconciliation Commission, Canada has a duty to ensure that Indigenous perspectives lead any planning process that aims to change land use in response to climate change in the North (Truth and Reconciliation Commission of Canada 2015).
Implications and future research
It is essential to acknowledge that the results presented here as potentially suitable for growing soy, corn, potato, and wheat are not a formalized prediction of these crops’ true frontiers. The agricultural frontiers identified in this study simply represent a range of estimates of where cropland expansion might be possible. As noted in our Methods section, our analysis was limited by the availability of high-resolution geospatial data for several important regulatory abiotic and biotic factors. Similarly, we made no effort to estimate the costs or returns of actually running farms in these regions. Such assessments are outside of the scope of this study, and here we were motivated to present the scientific and policy community with a sense of both the potential opportunities but also the possible consequences of developing agriculture in the North. All of this, however, suggests that a further step in this research program would be to sharpen up many aspects of this study such that it becomes a possible tool for planning to help lead to culturally appropriate economic development as well as sustainable environmental management.
In terms of improving the environmental aspects of this research, more data on soils is an obviously important element that should be developed. More specifically, thin acid soils broadly characterize large regions of the country’s boreal and tundra biomes, bedrock outcroppings with nutrient-poor peatlands often widely interspersed in low laying regions (Ecological Stratification Working Group 1995). The high nutrient demands of crops like corn and the deeper rooting structure of tubers like potatoes may prevent these crops from establishing under such harsh soil conditions—or may mean any such crops degrade the soil within a small number of harvests—without targeted remedial efforts (Ali et al. 2019). Local topography governing drainage patterns could further limit the agriculture frontiers of the row crops considered here, as local conditions generate extremes in soil moisture beyond a given crop’s tolerance. Intensive irrigation or drainage efforts could be enacted to support stable food productions from these regions, but this would have additional ecological impacts that require further study (Wichelns and Oster 2006; Sands et al. 2008).
Local near-surface permafrost extent and ice content also present a unique limitation to northern agricultural frontiers that need to be carefully studied and assessed. While near-surface permafrost remains intact, it would limit the seasonally thawed soils available for cultivation. However, the same warming conditions that enable northern agriculture may also lead to widespread permafrost thawing (Biskaborn et al. 2019). The loss of local permafrost, particularly ice-rich permafrost, can dramatically alter local hydrological flows, in extreme cases triggering rapid lake formation or drainage (Marsh and Neumann 2002). Unstable hydrological flows would challenge the possibility of agricultural development, perhaps necessitating highly dynamic cropping practices. What is more, permafrost is often critical for soil structure. Permafrost thaw can destabilize the soil matrix, triggering land subsidence and thaw slumping (Jones et al. 2019), presenting an additional barrier to long-term agricultural development.
The loss of local permafrost, particularly ice-rich permafrost, can dramatically alter local hydrological flows, in extreme cases triggering rapid lake formation or drainage (Marsh and Neumann 2002). Unstable hydrological flows would challenge the possibility of agricultural development, perhaps necessitating highly dynamic cropping practices. What is more, permafrost is often critical for soil structure. Permafrost thaw can destabilize the soil matrix, triggering land subsidence and thaw slumping (Jones et al. 2019), presenting an additional barrier to long-term agricultural development. The effectiveness of best management practices should be integrated into related future research, as adverse effects on water quality, quantity, and biodiversity can be effectively mitigated with best management practices (Power 2010). Regardless of the specifics, these regulatory factors across Canada’s North will undoubtedly further constrain the future agricultural frontiers and represent a critical knowledge gap for informed, sustainable northern development (Biskaborn et al. 2019).
Even if the analysis presented here overestimates the potential for agricultural expansion, our results still suggest that massive areas of Canada’s North will become suitable for growing soy, corn, potato and wheat in the future. In this way, these results contribute to the broad area of food security literature (Turner et al. 2003; Fedoroff et al. 2010; Foley et al. 2011; Steffen et al. 2015; Fraser et al. 2016; KC and Fraser 2017; KC et al. 2018). The potential degradation of ecosystem services highlighted in this paper is also all upper bound estimates. However, the magnitude of impacts identified, and the potential for very significant feedbacks in terms of environmental problems, should trigger concern. There is also some cautionary dialogue suggesting that further research is needed to understand the impacts of agricultural frontiers on food security and development in the north (e.g., Ray et al. 2013). Nevertheless, it is important to note that our analysis shows that climate change may make it possible for agriculture to expand to almost 75% of the northern region of Canada in the future.
Finally, we must acknowledge the limitation in how we have analyzed the implications of CCDAFs on Indigenous sovereignty. Our source for delineating Indigenous territory is Natural Resource Canada’s Aboriginal Lands of Canada Legislative Boundaries. These boundaries do not represent the entirety of Indigenous territory within the state of Canada, only territories that the Crown has formally acknowledged. Within these acknowledged territories and unceded and contested territories, the Crown has several obligations to Indigenous Peoples pertinent to CCDAFs as a signatory to the UNDRIP and section 35 of the Canadian Constitution. These obligations include the duty to engage with Indigenous communities under Free, Prior, and Informed Consent; addressing the right to Indigenous self-determination and self-governance; and the reassertion of the Indigenous–Crown relationship as nation-to-nation rather than nation-to-stakeholder (United Nations General Assembly 2008). Planning for and utilizing CCDAFs must follow procedures that satisfy Canada’s constitutional and UNDRIP obligations. Research, planning, and policy must be conducted through the lens of decolonization, otherwise CCDAFs may become a novel frontier for neo-colonial processes.
Therefore, future research will be necessary to ensure that Indigenous Nations and communities lead any development of CCDAFs and ensure that Indigenous sovereignty is being upheld through any land-use planning designed to take advantage of these agricultural frontiers. Research should be directed to empower Indigenous researchers, supporting them in assessing how Indigenous food systems will be affected by CCDAFs. Traditional food systems in regions like the Northwest Territories depend on the health of the land and water; hence, any agricultural expansion that utilizes trade-offs of ecosystem services that support traditional food systems can undermine northern food security by reducing the abundance of and access to traditional foods such as fish and caribou. Conversely, CCDAFs may support northern food security through nontraditional food systems by reducing transportation distances between growers and consumers and providing opportunities to increase incomes. These trade-offs of provisioning ecosystem services should be assessed thoroughly in future Indigenous-led research to alleviate food insecurity in Northern Canada.
Conclusion
Today, conventional agricultural development in the North is already undergoing considerable expansion. For example, in Northern Ontario, policymakers are prioritizing the conversion of Crown land to agricultural use as an economic diversification strategy (OMAFRA 2016). This strategy intends to take advantage of already increasing temperatures by expanding crop production acreage northward. For example, in Northern Ontario’s Kapuskasing area, the acreage of silage corn has increased by 51% since 2011 in response to a 26% increase in crop heat units (OMAFRA 2016). The Northwest Territories are similarly preparing to increase their presence in Canadian agriculture. In 2017, they launched their first-ever agricultural strategy to encourage the development of legislative tools and investments that will bolster local production of food as agricultural frontiers become realized (GWNT 2017).
In conclusion, this paper demonstrates that climate change will open opportunities for expanding farmland in the Northern region of Canada. This suggests the possibility of creating jobs and even reducing food insecurity in remote areas of Canada, where a lack of economic opportunities has created epidemic levels of food insecurity. However, there is a substantial risk of severe social and environmental impacts associated with the unregulated development of potential frontiers. Therefore, the development of expanding agricultural policies should engage with local communities and stakeholders to establish participatory processes that would ensure that economic development plans are led locally and that local communities are the primary beneficiaries of any land-use change.
Acknowledgements
This work was supported by the NSERC Strategic Network and Food from Thought Program at the University of Guelph, funded by the Canada First Research Excellence Fund.
References
Agriculture and Agri-Food Canada (AAFC) [dataset]. 2018. Annual space- based crop inventory for Canada, 2018. Agroclimate, geomatics and earth observation division, science and technology branch. [online]: Available from open.canada.ca/data/en/dataset/ba2645d5-4458-414d-b196-6303ac06c1c9.
Al-Anbari M, Thameer M, and Al-Ansari N. 2018. Landfill site selection by weighted overlay technique: Case study of Al-Kufa, Iraq. Sustainability Basel, Switzerland, 10(4): 999.
Ali W, Nadeem M, Ashiq W, Zaeem M, Thomas R, Kavanagh V, et al. 2019. Forage yield and quality indices of silage-corn following organic and inorganic phosphorus amendments in podzol soil under boreal climate. Agronomy, 9(9): 489.
Awawdeh M, ElMughrabi M, and Atallah M. 2018. Landslide susceptibility mapping using GIS and weighted overlay method: A case study from North Jordan. Environmental Earth Sciences, 77(21), 1–15.
Basharat M, Shah H, and Hameed N. 2016. Landslide susceptibility mapping using GIS and weighted overlay method: A case study from NW Himalayas, Pakistan. Arabian Journal of Geosciences, 9(4)|: 1–19.
Biskaborn BK, Smith SL, Noetzli J, Matthes H, Vieira G, Streletskiy DA, et al. 2019. Permafrost is warming at a global scale. Nature Communications, 10: 264
Bootsma T. 2013. Decadal Trends in Crop Heat Units for Ontario and Quebec from 1951 to 2010. [online]: Available from agrireseau.net/Agroclimatologie/documents/Ontario%20Quebec%20CHU%20trends%202012.pdf.
Conrad O, Bechtel B, Bock M, Dietrich H, Fischer E, Gerlitz L, et al. 2015. System for Automated Geoscientific Analyses (SAGA) v. 2.1.4. Geoscientific Model Development, 8(7): 1991–2007.
Council of Canadian Academies. 2014. Aboriginal Food Security in Northern Canada: An Assessment of the State of Knowledge. Council of Canadian Academies/ Conseil des academies canadiennes, Ottawa, ON.
Crowther TW, Todd-Brown KEO, Rowe CW, Wieder WR, Carey JC, Machmuller BL, et al. 2016. Quantifying global soil carbon losses in response to warming. Nature, 540: 104–108.
Davidson EA, and Ackerman IL. 1993. Changes in soil carbon inventories following cultivation of previously untilled soils. Biogeochemistry, 20: 161–193.
Ecological Stratification Working Group. 1995. A National Ecological Framework for Canada. Agriculture and Agri-Food Canada, Research Branch, Centre for Land and Biological Resources Research and Environment Canada, State of the Environment Directorate, Ecozone Analysis Branch, Ottawa/Hull. Report and national map at 1:7500 000 scale. [online]: Available from sis.agr.gc.ca/cansis/publications/manuals/1996/A42-65-1996-national-ecological-framework.pdf.
Environment and Climate Change Canada. 2021. Greenhouse gas emissions in Canada. [online]: Available from canada.ca/en/environment-climate-change/services/environmental-indicators/greenhouse-gasemissions.html.
FAO/IIASA/ISRIC/ISS-CAS/JRC. 2009. Harmonized World Soil Database (version 1.1). FAO, Rome, Italy and IIASA, Laxenburg, Austria. [online]: Available from fao.org/soils-portal/data-hub/soil-maps-and-databases/harmonized-world-soil-database-v12/en/.
Fedoroff NV, Battisti DS, Beachy RN, Cooper PJM, Fischhoff DA, Hodges CN, et al. 2010. Radically rethinking agriculture for the 21st century. Science, 327: 833–834.
Festa-Bianchet M, Ray JC, Boutin S, Côté SD, and Gunn A. 2011. Conservation of caribou (Rangifer tarandus) in Canada: an uncertain future. Canadian Journal of Zoology, 89(5): 419–434.
Fick SE, and Hijmans RJ. 2017. WorldClim 2: New 1-km spatial resolution climate surfaces for global land areas. International Journal of Climatology, 37: 4302–4315.
Foley JA, Ramankutty N, Brauman KA, Cassidy ES, Gerber JS, Johnston M, et al. 2011. Solutions for a cultivated planet. Nature, 478: 337–342.
Food and Agriculture Organization of the United Nations. 2021a. Crop Water Information. [online]: Available from fao.org/land-water/databases-and-software/crop-information/en/
Food and Agriculture Organization of the United Nations. 2021b. Catalogue of crops used in Bioenergy and Food Security (BEFS) Rapid Appraisal. [online]: Available from fao.org/3/bp841e/bp841e.pdf.
Fraser E, Legwegoh A, KC KB, CoDyre M, Dias G, Hazen S, et al. 2016. Biotechnology or organic? Extensive or intensive? Global or local? A critical review of potential pathways to resolve the global food crisis. Trends in Food Science & Technology 48: 78–87.
Government of Canada. 2020a. Aboriginal lands of canada legislative boundaries. [online]: Available from open.canada.ca/data/en/dataset/522b07b9-78e2-4819-b736-ad9208eb1067.
Government of Canada. 2020b. Regional, national and international climate modeling. In Natural Resources Canada. [online]: Available from cfs.nrcan.gc.ca/projects/3/9.
Government of Canada. 2021. Canadian protected and conserved areas database. [online]: Available from canada.ca/en/environment-climate-change/services/national-wildlife-areas/protected-conserved-areas-database.html#toc1.
GWNT. 2017. Northwest Territories (Nwt) Climate Change Strategic Framework.
Hannah L, Patrick R, KC KB, Fraser EDG, Donatti C, Saenz L, et al. 2020a. The Environmental Consequences of Climate-Driven Agricultural Frontiers. PLoS ONE, 15(2): e0228305.
Hannah L, Patrick R, KC KB, Fraser EDG, Donatti C, Saenz L, et al. 2020b. Crop suitability 3 methods. Figshare. [online]: Available from figshare.com/articles/Crop_Suitability_3Method_zip/11633352.
Hengl T, Mendes de Jesus J, Heuvelink GBM, Ruiperez Gonzalez M, Kilibarda M, Blagotic A, Shangguan W, et al. 2012. SoilGrids250m: Global gridded soil information based on machine learning. PLoS ONE. 12: e.0169748.
Hijmans J. 2020. raster: Geographic data analysis and modeling. R package version 3.4-5. [online]: Available from CRAN.R-project.org/package=raster.
Hijmans RJ, Cameron SE, Parra JL, Jones PG, and Jarvis A. 2005. Very high resolution interpolated climate surfaces for global land areas. International Journal of Climatology, 25 (15): 1965–1978.
IPCC. 2014. Climate Change 2014: Synthesis Report. In Contribution of Working Groups I, II and III to the Fifth Assessment Report of the Intergovernmental Panel on Climate Change. Edited by Core Writing Team, RK Pachauri and LA Meyer IPCC, Geneva, Switzerland. 151 pp.
Jones MKW, Pollard WH, and Jones BM. 2019. Rapid initialization of retrogressive thaw slumps in the Canadian high Arctic and their response to climate and terrain factors. Environmental Research Letters, 14: 115008.
Kaliraj S, Chandrasekar N, and Magesh N. 2015. Evaluation of multiple environmental factors for site-specific groundwater recharge structures in the Vaigai River upper basin, Tamil Nadu, India, using GIS-based weighted overlay analysis. Environmental Earth Sciences, 74(5): 4355–4380.
KC KB, and Fraser EDG. 2017. Strategies to boost global food production: Modelling socioeconomic policy scenarios. Cogent Food & Agriculture, 3(1): 1309739.
KC KB, Dias GM, Veeramani A, Swanton CJ, Fraser D, Steinke D, et al. 2018. When too much isn’t enough: Does current food production meet global nutritional needs? PLoS ONE, 13(10): e0205683.
KC KB, Montocchio D, Berg A, Fraser EDG, Daneshfar B, and Champagne C. 2020. How climatic and sociotechnical factors influence crop production: a case study of canola production. SN Applied Sciences, 2: 2063.
King M, Altdorff D, Li P, Galagedara L, Holden J, and Unc A. 2018. Northward shift of the agricultural climate zone under 21 st -century global climate change. Scientific Reports, 8(1): 1–10.
Lal R. 2002. Soil carbon dynamics in cropland and rangeland. Environmental Pollution, 116(3): 353–362.
Laurance WF, Sayer J, Cassman KG. 2014. Agricultural expansion and its impacts on tropical nature. Trends in Ecology & Evolution 29: 107–116.
Lawrence DM, Koven CD, Swenson SC, Riley WJ, and Slater AG. 2015. Permafrost thaw and resulting soil moisture changes regulate projected high-latitude CO2 and CH4 emissions. Environment Research Letter, 10: 094011.
Li G, Zhang X, Cannon AJ, Murdock T, Sobie S, Zwiers F, et al. 2018. Indices of Canada’s future climate for general and agricultural adaptation applications. Climatic Change, 148(1–2): 249–263.
Marsh P, and Neumann NN. 2002. Processes controlling the rapid drainage of two ice-rich permafrost-dammed lakes in NW Canada. Hydrological processes, 15(18): 3433–3446.
McKenney DW, Hutchinson MF, Papadopol P, Lawrence K, Pedlar J, Campbell K. et al. 2011. Customized spatial climate models for North America. Bulletin of the American Meteorological Society, 92(12): 1611–1622. [online]: Available from journals-ametsoc-org.subzero.lib.uoguelph.ca/view/journals/bams/92/12/2011bams3.
Molotoks A, Kuhnert M, Dawson TP, Smith P. 2017. Global hotspots of conflict risk between food security and biodiversity conservation. Land, 6(4): 67.
Mosby I, Rotz S, and Fraser E. 2020. Uncertain Harvest: The future of food on a warming planet. University of Reginal Press, Regina.
Natali SM, Schuur EAG, Mauritz M, Schade JD, Celis G, Crummer KG, et al. 2015. Permafrost thaw and soil moisture driving CO2 and CH4 release from upland tundra. Journal of Geophysical Research: Biogeosciences, 120: 525–537.
Natural Resources of Canada. 2020. Leading species grids. [online]: Available from ftp://ftp.nrcan.gc.ca/pub/outgoing/Swystun/primary_species.7z.
New Brunswick Canada. 2021. Land development. [online]: Available from www2.gnb.ca/content/gnb/en/departments/10/agriculture/content/land_development.html
NWT Bureau of Statistics. 2014. Food Security (Population 12 Years and Older): Northwest Territories and Canada, 2003-2014. Statistics Canada, Canadian Community Health Survey (CCHS).
OMAFRA. 2016. Northern ontario’s food strategy [online]: Available from files.ontario.ca/mnrf-applicants-guide-apply-for-crown-land-agri-purpose-northern-on.pdf.
Ontario Ministry of Agriculture, Food and Rural Affairs. 2021. Classifying prime and marginalagricultural soils and landscapes: Guidelines for application of the Canada land inventory in Ontario. [online]: Available from omafra.gov.on.ca/english/landuse/classify.htm.
Parry ML, Rosenzweig C, Iglesias A, Livermore M, and Fischer G. 2004. Effects of climate change on global food production under SRES emissions and socioeconomic scenarios. Global Environmental Change 14 (1): 53–67.
Palomo I. 2017. Climate Change Impacts on Ecosystem Services in High Mountain Areas: A Literature Review. Mountain Research and Development, 37: 179–187.
Phelps J, Carrasco LR, Webb EL, Koh LP, and Pascual U. 2013. Agricultural intensification escalates future conservation costs. Proceedings of the National Academy of Sciences of the United States of America 110: 7601–7606.
Popp A, Calvin K, Fujimori S, Havlik P, Humpenoder F, Stehfest E, et al. 2017. Land-use futures in the shared socioeconomic pathways. Global Environmental Change, 42: 331–345.
Power EM. 2008. Conceptualizing food security for Aboriginal people in Canada. Canadian Journal of Public Health/Revue Canadienne de Sante Publique, 95–97.
Ray DK, Muller ND, West PC, and Foley JA. 2013. Yield Trends Are Insufficient to Double Global Crop Production by 2050. PLoS ONE, 8: e66428.
Sands GR, Song I, Busman LM, and Hansen BJ. 2008. The effects of subsurface drainage depth and intensity on nitrate loads in the Northern Cornbelt. Transactions of the ASABE. 51(3): 937–946.
Statistics Canada. 2016. Census boundary files [online]: Available from www12.statcan.gc.ca/census-recensement/2011/geo/bound-limit/bound-limit-2016-eng.cfm.
Statistics Canada. 2020. Road Network File, 2020. Statistics Canada Catalogue no. 92-500-X. [online]: Available from www12.statcan.gc.ca/census-recensement/2011/geo/RNF- FRR/index-2011-eng.cfm?year=16.
Steffen W, Richardson K, Rockström J, Cornell SE, Fetzer I, Bennett EM, et al. 2015. Planetary boundaries: Guiding human development on a changing planet. Science, 347(6223): 1259855.
Sys C, Van Ranst E, Debaveye J, and Beernaert F. 1993. Land Evaluation Part III, Crop Requirements; Agricultural Publications No. 7. ITC Ghent, Ghent, Belgium.
Tashayo B, Honarbakhsh A, Akbari M, and Eftekhari M. 2020. Land suitability assessment for maize farming using a GIS-AHP method for a semi- arid region, Iran. Journal of the Saudi Society of Agricultural Sciences, 19(5): 332–338.
Tilman D. 1999. Global environmental impacts of agricultural expansion: The need for sustainable and efficient practices. Proceedings of the National Academy of Sciences of the United States of America 96: 5995–6000.
Truth and Reconciliation Commission of Canada. 2015. [online]: Available from trc.ca/assets/pdf/Honouring_the_Truth_Reconciling_for_the_Future_July_23_2015.pdf.
Turetsky MR, Benscoter B, Page S, Rein G, Vander GR, and Watts A. 2015. Global vulnerability of peatlands to fire and carbon loss. Nature Geoscience, 8: 11–14.
Turner BL, Kasperson RE, Matson PA, McCarthy JJ, Corell RW, Christensen L, et al. 2003. A framework for vulnerability analysis in sustainability science. Proceedings of the national academy of sciences, 100(14): 8074–8079.
United Nations General Assembly. 2008. United nations declaration on the rights of indigenous peoples. [online]: Available from un.org/esa/socdev/unpfii/documents/DRIPS_en.pdf.
Vázquez-Quintero G, Prieto-Amparán J, Pinedo-Alvarez A, Valles-Aragón M, Morales-Nieto C, Villarreal-Guerrero F. et al. 2019. GIS-Based multicriteria evaluation of land suitability for grasslands conservation in chihuahua, Mexico. Sustainability (Basel, Switzerland), 12(1): 185.
Walker XJ, Baltzer JL, Bourgeau-Chavez L, Day NJ, Dieleman C, Johnstone JF, et al. 2020. Patterns of Ecosystem Structure and Wildfire Carbon Combustion Across Six Ecoregions of the North American Boreal Forest. Frontires in . Forests and. Global. Change, 3: 87.
Wang L, and Liu H. 2006. An efficient method for identifying and filling surface depressions in digital elevation models for hydrologic analysis and modelling. International Journal of Geographical Information Science, 20(2): 193–213.
Watson JEM. 2014. Human responses to climate change will impact biodiversity conservation: It’s time we start planning for them. Conservation Letters, 7: 1–2.
Wichelns D, and Oster JD. 2006. Sustainable irrigation is necessary and achievable, but direct costs and environmental impacts can be substantial. Agricultural Water Management 86(1–2): 114–127.
World Bank. 2021. Data catalog [online]: Available from datacatalog.worldbank.org/dataset/world-bank-official-boundaries/resource/12cc761c-bb67-45a7-82ff-eb64b67bdbdc (https://datacatalog.worldbank.org/public-licenses#cc-by)
Zabel F, Putzenlechner B, and Mauser W. 2014. Global agricultural land resources – a high resolution suitability evaluation and its perspectives until 2100 under climate change conditions. PLoS ONE, 9(9): e107522.
Supplementary materials
Supplementary Material 1 (DOCX / 31.5 KB)
- Download
- 31.53 KB
Supplementary Material 2 (PDF / 32 MB)
- Download
- 32.10 MB
Information & Authors
Information
Published In
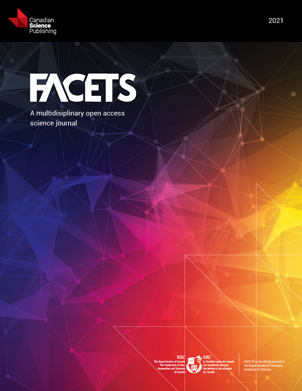
FACETS
Volume 6 • Number 1 • January 2021
Pages: 1728 - 1752
Editor: Elena M. Bennett
History
Received: 29 October 2020
Accepted: 3 August 2021
Version of record online: 21 October 2021
Notes
This paper is part of a Collection titled “Food, Fiber, Fuel, and Function: Pathways to manage ecosystem services in Canada’s working landscapes”.
Copyright
© 2021 KC et al. This work is licensed under a Creative Commons Attribution 4.0 International License (CC BY 4.0), which permits unrestricted use, distribution, and reproduction in any medium, provided the original author(s) and source are credited.
Data Availability Statement
All relevant data are within the paper and in the Supplementary Material.
Key Words
Sections
Subjects
Authors
Author Contributions
KBK and EDGF conceived and designed the study.
KBK, DW, and VG performed the experiments/collected the data.
KBK, DW, and VG analyzed and interpreted the data.
All contributed resources.
All drafted or revised the manuscript.
Competing Interests
Canada’s North: An ecosystem service perspective.
Metrics & Citations
Metrics
Other Metrics
Citations
Cite As
Krishna Bahadur KC, Arthur Gill Green, Dan Wassmansdorf, Vivek Gandhi, Khurram Nadeem, and Evan D.G. Fraser. 2021. Opportunities and trade-offs for expanding agriculture in Canada’s North: an ecosystem service perspective. FACETS.
6: 1728-1752.
https://doi.org/10.1139/facets-2020-0097
Export Citations
If you have the appropriate software installed, you can download article citation data to the citation manager of your choice. Simply select your manager software from the list below and click Download.
Cited by
1. Socioecological dynamics of diverse global permafrost-agroecosystems under environmental change
2. Simultaneous multi-crop land suitability prediction from remote sensing data using semi-supervised learning
3. The interactions among landscape pattern, climate change, and ecosystem services: progress and prospects
4. Little brown bat activity patterns and conservation implications in agricultural landscapes in boreal Yukon, Canada
5. Assessment of soil organic carbon stocks in Alberta using 2-scale sampling and 3D predictive soil mapping
6. Climate change creates opportunities to expand agriculture in the Hindu Kush Himalaya but will cause considerable ecosystem trade-offs
7. Horizon scanning and review of the impact of five food and food production models for the global food system in 2050
8. Managing Canada’s land- and seascapes for multiple ecosystem services in the Anthropocene: introduction to the Food, Fiber, Fuel, and Function collection