The many pathways of climate change affecting coastal ecosystems: a case study of western Vancouver Island, Canada
Abstract
Climate change threatens marine ecosystems with known effects on marine life, including changes in metabolic rates, survival, and community structure. Based on a structured literature review, we developed a conceptual “pathways of effects” model that summarizes how three stressors associated with climate change (warming, acidification, and storms) affect functional species groups on the West Coast of Vancouver Island, Canada. We identified 155 distinct pathways from the three stressors through 12 categories of biological effects ranging from changes in the biochemistry of individual organisms to effects on community composition. Most species groups were affected by several climate stressors and via many pathways, although individual studies generally considered only a small fraction of relevant pathways. These effects depended on the species of interest and geographical location, highlighting the importance of local research. Climate change stressors exert complex, sometimes contradictory effects that vary across ecological scales. For example, some stressors that adversely affected a species in laboratory studies appeared beneficial in community-scale field studies. Pathways of effects models are helpful tools to summarize scientific studies across ecological scales. Compiling them in standardized databases would allow researchers and practitioners to search across species and regions to better support ecosystem-based management and environmental impact assessment.
1. Introduction
Climate change threatens the biodiversity, function, and services provided by coastal ecosystems (Cooley et al. 2022). Important climate stressors include warming, acidification, increased storm frequency, sea level rise, deoxygenation, and changes to stratification, circulation, connectivity, and upwelling (Brierley and Kingsford 2009). These environmental changes affect marine life in many ways and across ecological scales (Harley et al. 2006; Hoegh-Guldberg and Bruno 2010). For example, temperature affects the metabolic rates, reproduction, and behaviour of various species (Chen and Irvine 2001; Szathmary et al. 2009; Starko et al. 2022) and storms can dislodge seaweeds (Burnett and Koehl 2022) and transport toxic algal blooms (McCabe et al. 2016). Together, such effects can drive significant changes at the ecosystem scale, which have already been observed worldwide (Poloczanska et al. 2013).
While many laboratory and field studies shed light on stressor effects on individual species or local communities (Crain et al. 2008; Darling and Côté 2008; Côté et al. 2016), translating these empirical results into a holistic view of potential ecosystem-scale changes remains a challenge (Borja et al. 2020; Bass et al. 2021). Here, we demonstrate the use of conceptual pathways of effects models to integrate scientific results across studies and ecological scales. Using the West Coast of Vancouver Island as a case study, we ask: How are climate stressors known to affect key functional groups of coastal species from this region? Which pathways of effects are supported by local studies? Do studies at different ecological scales (e.g., laboratory studies of single species vs. field studies of whole communities) come to similar conclusions?
Pathways of effects models are directed graphs that link human activities and the stressors they cause to known effects on chosen endpoints (e.g., species of interest). In pathways of effects models, each pathway is based on peer-reviewed literature or expert opinion about how stressors affect ecosystem components. Such models are often used by governmental organizations to support environmental risk analyses (e.g., Government of Canada 2012; Brownscombe and Smokorowski 2021). They can focus on specific human activities (e.g., commercial shipping: Hannah et al. 2020), species and habitats (e.g., fish and fish habitats: Brownscombe and Smokorowski 2021; killer whales: Murray et al. 2019), or environmental issues in a specific geographical area (Pickard et al. 2015). In scientific applications, pathways of effects models are a promising, but underutilized tool for informing quantitative, ecosystem-scale models of human impacts (Stock et al. 2023). For example, Murray et al. (2021) developed a pathways of effects model for multiple stressor effects on killer whales, which then informed a quantitative population viability analysis.
Here, we describe a pathways of effects model for the West Coast of Vancouver Island, one of the most productive marine ecosystems in Western North America (Ware and Thomson 2005; Gregr et al. 2020). Climate change is one of the main threats to this coastal ecosystem now and in the future (Murray et al. 2015). Consequently, concise information on potential climate change effects on the coastal biota of the region is needed for science-based management and planning. By presenting a pathways of effects model summarizing the current state of knowledge on climate change effects on this ecosystem, we make three contributions. First, we provide a summary of knowledge about the species-level effects of climate change on the coastal biota of the West Coast of Vancouver Island. Such regional knowledge is critical because marine conservation, management, and planning often occur at local to regional extents and must be based on understanding the particular ecosystem and its species (Crowder and Norse 2008; Cavallo et al. 2019). Second, we argue that understanding how people affect marine ecosystems must consider multiple pathways for each stressor. Third, we show how pathways of effects models can help summarize mechanisms through which complex human impacts occur and outline how the future development of pathways of effects databases might support regional environmental research and management.
2. Methods
2.1. Study region
As a case study, we chose the coastal marine ecosystem off the west coast of Vancouver Island, Canada, a highly productive temperate region of the North Pacific. Strong seasonality of oceanographic conditions and the influence of the California Current in the south and the Alaska Current in the north create a dynamic and biodiverse coastal environment (Crawford and Thomson 1991; Mackas et al. 2001). Stressors driven by global climate change are altering the biophysical conditions of the area. For example, regional summer sea surface temperature increased by 0.67–0.78 °C from 1935 to 2016 and continues to rise (Iwabuchi and Gosselin 2019). Persistent marine heatwaves occurred in the northeast Pacific from 2014–2016 to 2019–2020 (DiLorenzo and Mantua 2016; Chen et al. 2021). In June 2021, a shorter but intense heatwave caused mass mortality of intertidal species across southwestern Canada (Philip et al. 2022; Raymond et al. 2022). Ocean acidification also poses risks to recreationally and commercially fished and cultured species in British Columbia—especially in combination with other climate stressors like warming and deoxygenation (Haigh et al. 2015).
2.2. Literature review and pathways of effects modelling
We used structured literature review methods to develop the pathways of effects model, focusing on the effects of three stressors (warming, including heatwaves, acidification, and storms) on 22 functional species groups. We searched for literature in the Web of Science from 1900 to April 2022 for effects of warming and acidification, and from 1900 to April 2023 for effects of storms and heatwaves. We preferred studies specific to the West Coast of Vancouver Island and nearby North American Pacific locations, as described near the end of the Methods section.
A pathways of effects model links human activities (here: any activities that emit greenhouse gases, not shown in the model for simplicity) to the stressors they cause (here: warming, acidification, and storms), and their effects (in 12 categories, see below) on endpoints (the 22 species groups). The species groups were drawn from an existing quantitative food web model for the region (Gregr et al. 2020). This grouping focused on species that are commercially important, are prey for sea otters, or are trophically linked to those species. It was originally chosen to allow a quantification of the effects of sea otters on ecosystem services. We maintained this grouping because the same species retain commercial relevance for coastal communities of the West Coast of Vancouver Island and to create a foundation for potential later simulations of selected pathways with the existing food web model. We searched the literature for each group with keywords including its name and the scientific and common names of the most common species within the group. Contrary to Gregr et al. (2020), we included larval life stages of species in their respective adult-stage functional group instead of grouping them under zooplankton. In this way, we included climate effects on early life stages as potential effects on the recruitment of the species group.
To group effects into general categories, we first used the categories proposed by Busch and McElhany (2016). However, these categories did not allow an unambiguous classification of the literature from our search. We hence modified these effect categories substantially, resulting in the 12 categories listed in Table 1. Studies reporting an effect on population size without attributing this effect to changes in survival or reproduction and recruitment were classified in the effect category “Population size”. In contrast, studies reporting effects on “Survival” or “Reproduction and recruitment” were only classified in the respective category, not as effects on “Population size”, to avoid double-counting.
Table 1.
Effect category | Description | Examples of reported indicators and effects |
---|---|---|
Behavioural | Effects on an organism's behaviour, including anxiety, feeding, and reproductive behaviour | Diurnal movements, feeding rates, initiation of feeding, predation increase, prey selection, food preference, preference for dark zone (anxiety), total path length travelled, vertical distribution, number of mates per male |
Calcification | Effects on calcification and calcified body parts | Shell dissolution, shell strength, shell malformations and deformities, shell thickness, skeletal growth, shell area, shell size, calcium content, Mg/Ca ratio |
Community | Observed community changes within the functional group | Percentage of Pisaster in sea star community, species richness, fraction of southern species, fraction of northern species, proprotion of diatoms, proportion of nanoflagellates |
Disease/illness | Disease and exposure to toxins | Wasting disease, proportion of senescent (non-healthy) individuals, blade bleaching, toxicity of bloom, paralytic shellfish poisonings |
Genomic | Effects on genes, gene expression, evolution | Gene encoding for heat shock protein HSP 70, gene expression, genetic diversity, level of polymorphism, evolutionary potential, evolutionary response, holdfast resistance, rate of evolution, gene expression of carbonic anhydrase, transcriptome |
Geographical range and seasonality | Changes to where and when species occur | Range expansion, biogeographical shift, spatial distribution, suitable habitat, range, protected area connectivity, advection towards shore |
Growth | Effects on the growth rate, size, or weight of organisms | Biomass production rates, body length, body size, growth rate, fond and net blade growth rates, length at age, population recovery (growth), production, shell growth, primary productivity, shell size, size at 50% maturity |
Morphology | Changes to an organism's morphology, except changes in calcified body parts (→ Calcification) and reproductive organs (→ Reproduction/Recruitment) | Otolith size |
Physicochemical | Effects on physical and chemical composition and processes within an organism's body, including body temperature, metabolism, and proteomics | ATP production, body temperature, C:N ratio, carbon (d13C), d15N, expression of heat shock proteins HSP70 and HSP 71, oxygen consumption, oxygen consumption rate/metabolic rate, phenolitic content, pumping arrest, respiration rate, frequency of heart beat, HSP 90, maximum quantum yield of PSII, organic biomass, residence time (time taken to expel dye from the oscula), heart rate, hepatosomatic index, ingestion rate, proteomic profiles, pumping strength, tissue N content and C/N ratio, antioxidant capacity, filtration rate, NH4 + excretions, probability of tissue withdrawal, total lipids |
Population size | Changes in population size (if not attributed to reproduction, growth, or survival) | Abundance (of adults or youth-of-the-year), biomass, density, front density |
Reproduction/recruitment | Effects on reproduction, including effects on development of reproductive organs and completion of life cycle | Density of sporophytes, dispersal, gametogenesis, number of class 1 and class 2 oocytes, germination rate and success, gonad growth, hatching time, number of eggs, oocytes per unit area, pre-settlement growth rates, time to reach sexual maturity, settlement success, spawn indices, stock recruitment, survival of larvae, yolk sac area, zoeal duration (time of settlement), dormancy, egg production, embryo mortality, gonadal index, larval length, fecundity, gametophyte size, pelagic duration, percentage of spawners, gonosomatic index, settlement time, larval survival, zoeal mortality, zoeal survival, development of secondary sex structures, dry mass of embryo, eggs transferred per mating episode, germling growth rates, mortality of spores, reproduction rate, oocytes per follicle, percentage of individuals with sexually mature gonads |
Survival | Effects on survival/mortality | Abundance (if attributed to changes in survival), dislodgement, breakage, longevity, mortality rate, survival rate, lifespan |
We searched the literature for every combination of species and stressor using respective keywords. Every search began with a geographical location limited to Vancouver Island—e.g., TS = (“acidification” OR “ph”) AND TS = (“Geoduck” OR “Panopea generosa”) AND TS = (“Vancouver Island”). We read abstracts from every paper in the search results and scanned for relevance every paper containing at least one keyword from (1) the functional group, (2) the human activity, and (3) the geographical scope. If finding fewer than two relevant papers, we expanded the geographical scope by adding the keyword “British Columbia” [TS = (“Vancouver Island” OR “British Columbia”)]. If finding, again, fewer than two relevant papers, we expanded the search by adding the keyword “Pacific” [TS = (“Vancouver Island” OR “British Columbia” OR “Pacific”)]. We dropped the location search terms altogether if this search yielded less than two relevant results using all geographical keywords. In contrast, if finding too many relevant results (>80), we narrowed the search by adding descriptive keywords (e.g., adding “East*” to searches in “Pacific”).
This search process yielded papers specific to Vancouver Island or at least from California to Alaska for most functional groups, except for giant kelp. For effects of acidification and warming on this group, we included papers from Chile and New Zealand. We found no studies on the effects of acidification on giant kelp using location search terms and too many relevant studies when we did not include any geographical keywords. In this case, we reviewed the three most recent relevant and the three most widely read (by sorting them by all-time usage in the Web of Science) studies. Finally, we also reviewed papers cited in the search results and added them to the pathways of effects model if they were relevant to our study area and described new pathways.
In total, we conducted 88 keyword searches that yielded 1432 papers. Thereof, 184 were relevant after skimming and were reviewed in full. However, 59 studies had weak conclusions or found no effect on the functional group. We did not include such studies in the pathways of effects model, but discuss such findings in the supplementary materials. We identified one additional study that did not state its geographic location in the title, abstract, or keywords (Supratya et al. 2020) and was hence not found through the keyword search, but cited in a review of kelp research in the Salish Sea (Hollarsmith et al. 2022). Hence, 126 studies were included, seven of which considered more than one stressor. Because of the small number of studies considering several stressors, we discuss results on stressor interactions qualitatively.
For each study, we collected the following data: reference, functional group, life stage, stressor, effect category, study location, research design (e.g., lab vs. field experiments), and an “evidence statement” (a short excerpt copied from the study stating the described effect). For categories where the direction of the effect could be clearly defined (e.g., population size, reproduction), we also noted the direction of the effect. For geographical range, we defined an increase as species being reported to occur in new areas, a decrease as species disappearing from an area, and a change without direction as a reported shift in the species’ range that might encompass expansion and reduction in different places. For categories where increases and decreases could not be clearly defined (e.g., behaviour, morphology), we only noted the directionless change. We provide these data as supplementary materials.
3. Results
3.1. Pathways of effects model
Climate change effects on the West Coast of Vancouver Island ecosystem are complex: we found 155 pathways of effects in the literature (Fig. 1; Table 2)—155 different ways that climate change affects the species groups of this region. The Supplementary Materials contain a summary of the pathways and the evidence for them organized by species group.
Fig. 1.
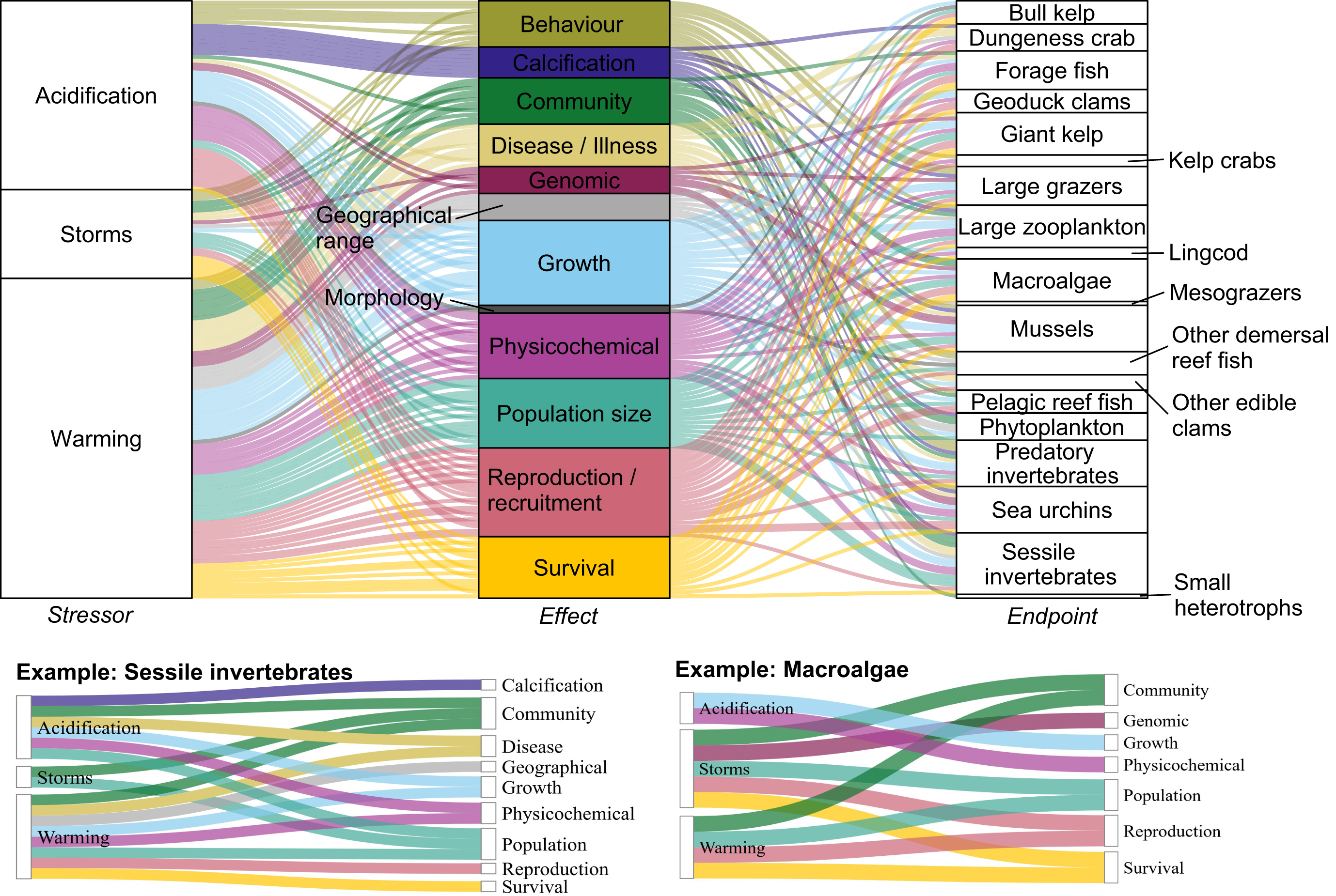
Table 2.
Most pathways were related to warming (54%), reflecting a predominance of articles on this stressor (see Section 3.2). Furthermore, most pathways came from a single study (66%) or two studies (22%). However, this reflects the geographical hierarchy of our literature search, and there is likely more evidence for many pathways if searching globally. Over half of the pathways came from studies specific to the West Coast of Vancouver Island (35%) or other places in British Columbia (23%). Most others came from studies from the West Coast of the USA (39%). Only 3% of pathways came from studies in other regions.
3.2. Literature statistics
There was evidence of climate change effects on most functional groups. Fifty-nine percent of articles included in the pathways of effects model studied warming, reporting effects on 19 of 22 species groups. Twenty-nine percent studied acidification, reporting effects on 14 groups. Seventeen percent studied storms, reporting effects on 13 groups. However, not all functional groups were studied equally: about 10% of articles were about large zooplankton, 10% about sessile invertebrates, 10% about predatory invertebrates, 10% about mussels, and another 10% about macroalgae. In contrast, the search yielded no relevant articles for sea otters and few studies for small grazers, which found no effects and are hence not included in the model. We found only one study for species from three other groups (small heterotrophs, small mezograzers, and lingcod).
The studies used various research approaches. Most were empirical, reporting field research (46%), laboratory research (35%), or a combination of both laboratory and field approaches (11%). Less common research designs included modelling (such as a stock recruitment model incorporating water temperature; Chen and Irvine 2001), studies of paleoanalogues (Hay et al. 2007), and one literature review (Burnett and Koehl 2022).
The availability of local literature varied across stressors and functional groups (Fig. 2). While local literature about the effects of warming existed for many functional groups, we found fewer local studies about the effects of acidification and storms. Hence, pathways for these stressors often came from studies conducted elsewhere, but mainly on the North American West Coast. Of the 126 studies reflected in the pathways of effects model, 29% were from the West Coast of Vancouver Island, 18% from elsewhere in British Columbia, 49% from the West Coast of the USA, and 4% from other locations. Because some studies reported several pathways, these percentages for studies differ from those for pathways in Section 3.1. Furthermore, because some studies investigated several stressors or functional groups, and some found several kinds of effects, reported percentages can add to >100%. Finally, the results above reflect that we only searched beyond our study region if too few local studies were found. They do not reflect the availability of literature globally.
Fig. 2.
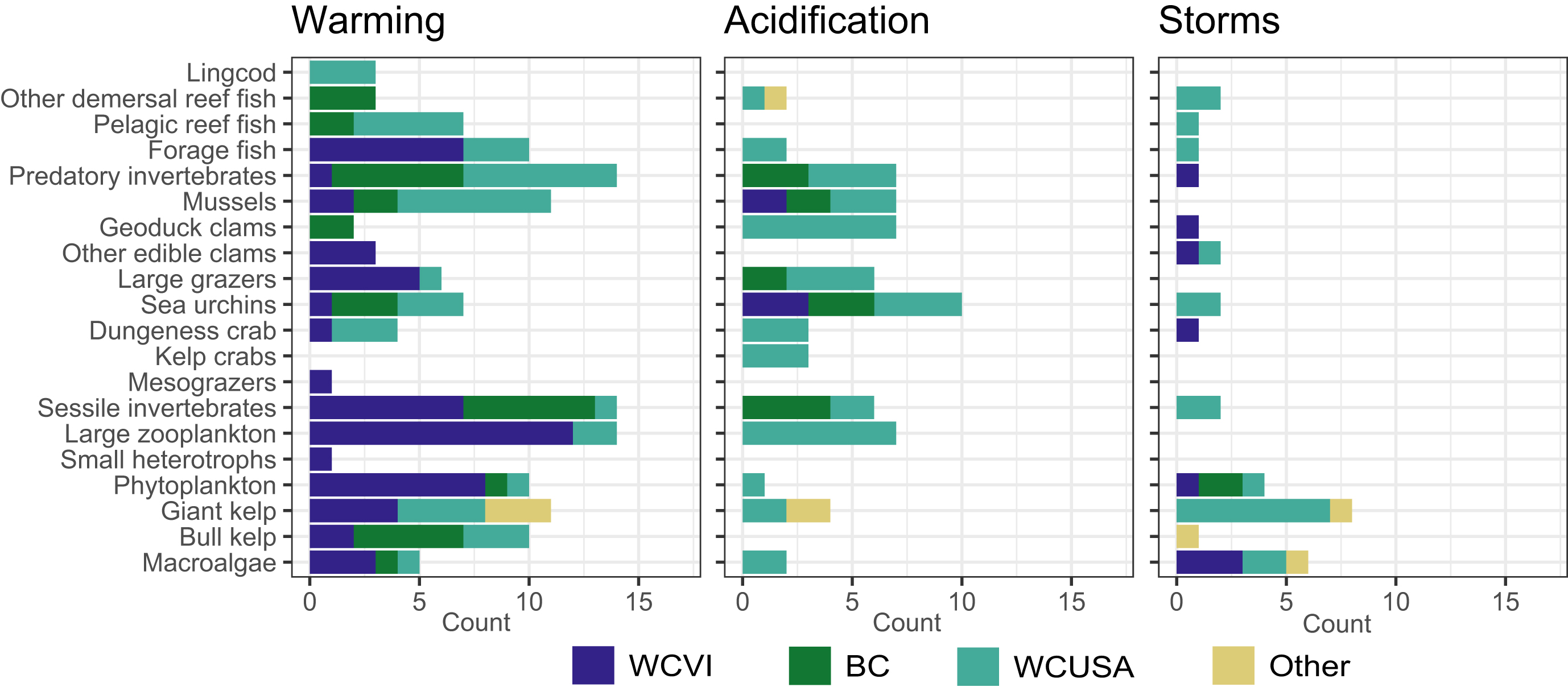
3.3. Multiple stressors and multiple effects
Most functional groups were affected by multiple stressors and multiple kinds of effects (Fig. 3). Ten functional groups were affected by all three stressors, six by two stressors, and four by one stressor (for two groups, sea otters and small grazers, we found no literature supporting effects of these stressors). Furthermore, for all groups except two, the literature described several types of effects, on average 5.7 and up to 9 (for large zooplankton, mussels, predatory invertebrates, and sea urchins). Accordingly, most functional groups were endpoints of several pathways, on average 7.6 and up to 14 (for sessile invertebrates). The only exceptions were small heterotrophs and mesograzers with only one type of effect, and sea otters and small grazers with no reported effects.
Fig. 3.
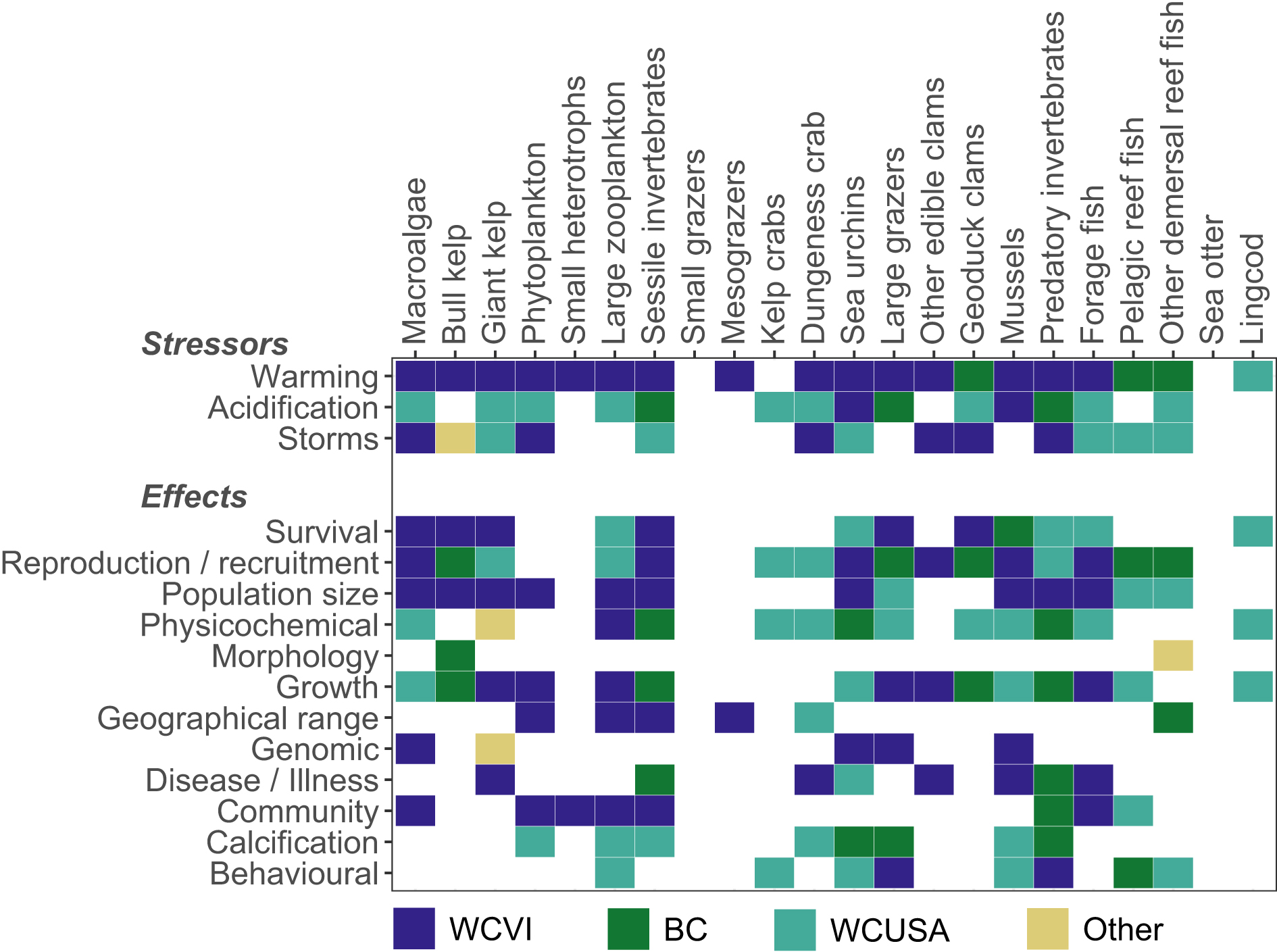
Only seven of the 126 papers investigated two stressors (Table 3). Four found significant interactions, but mostly weak and only for a subset of measured outcome variables. Hence, stressor interactions in the coastal ecosystem of our study region remain mostly unknown.
Table 3.
Reference | Stressors | Endpoint | Reported effects |
---|---|---|---|
Allen and Wolfe (2013) | Warming, storms | Phytoplankton | Warming and storms both influenced the seasonality of blooms. |
Villalobos et al. (2020) | Warming, acidification | Forage fish | Warming affected the mortality and metabolism of Pacific Herring. Acidification alone had only small effects. However, it exacerbated the effects of warming. |
Stevenson et al. (2020) | Warming, acidification | Sessile invertebrates | Warming and acidification, together and in combination, adversely affected pumping capacity, tissue withdrawal, and structural integrity. |
Padilla-Gamiño et al. (2013) | Warming, acidification | Sea urchins | Warming and acidification had mainly additive physicochemical and genomic effects. |
McCabe et al. (2016) | Warming, storms | Phytoplankton | Warming initiated a harmful algal bloom offshore. Storms transported it to nearshore ecosystems. |
Hollarsmith et al. (2020) | Warming, acidification | Giant kelp | The effects of warming and acidification differed between populations. Overall, warming negatively and acidification positively affected reproduction, with no significant interactions. |
Gaitán-Espitia et al. (2014) | Warming, acidification | Giant kelp | Warming and acidification had negative effects on reproduction. There was a significant interaction of these stressors affecting spore mortality and dormancy, but no significant interaction for germination rates. |
We distinguished the direction of effects for 102 of the 155 pathways (Fig. 4). For 68 of these pathways, the direction came from a single study (either because it was the only study reporting the effect or because other studies reported an effect but not a meaningful direction). For 24 pathways, two or more studies reported the effect and agreed on the direction. Warming and, to a lesser extent, acidification and storms were consistently associated with more disease and illness. Acidification consistently reduced calcification for phytoplankton, zooplankton, and invertebrates. Effects on growth, population size, survival, and reproduction were mainly but not always negative. For 10 pathways, two or more studies reported the same effect but with opposite directions.
Fig. 4.
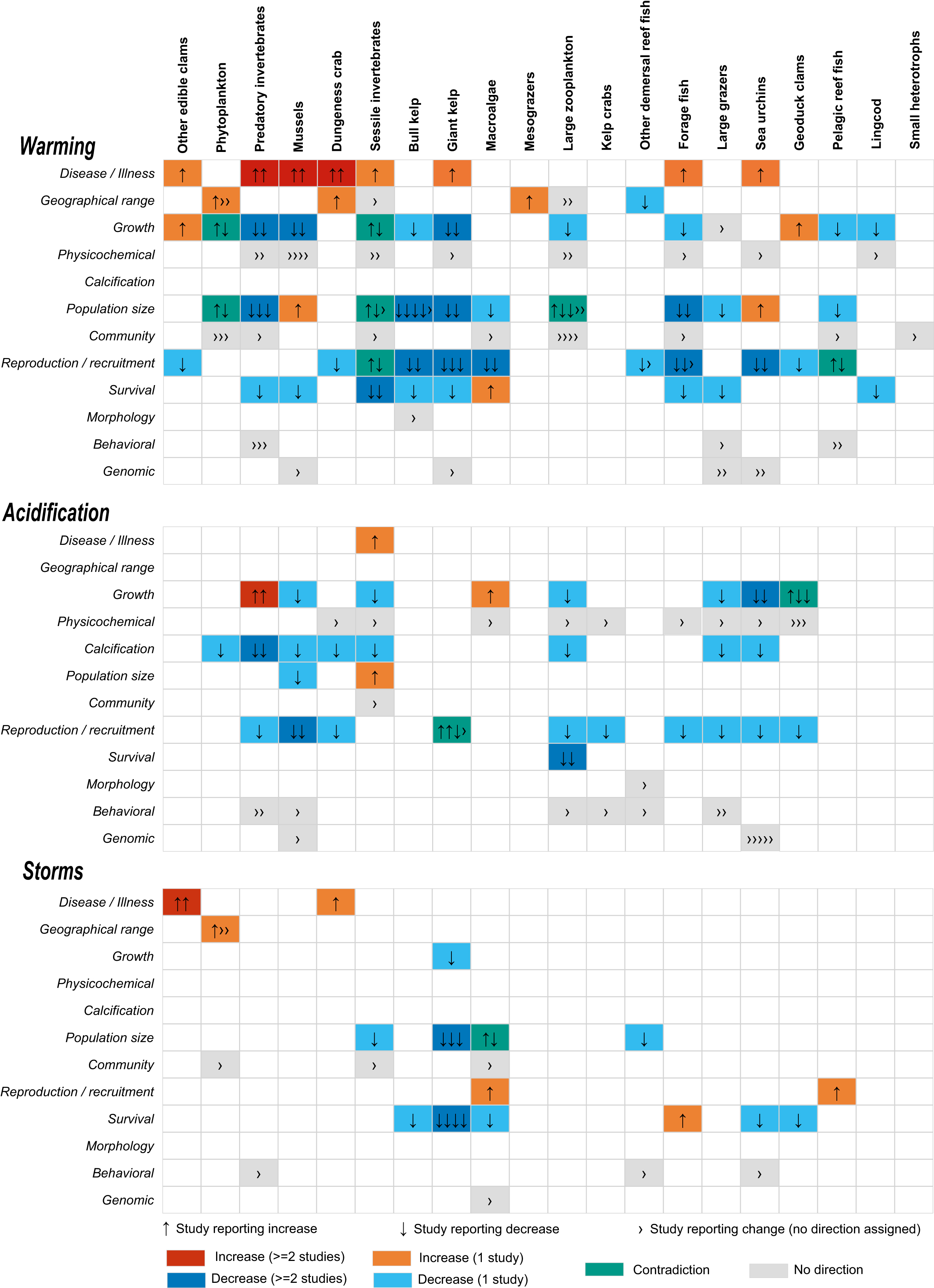
Taken together, the documented climate change effects were complex and sometimes contradictory. Stressors that negatively affect one species group might directly or indirectly benefit another. For example, storms can dislodge giant kelp and sweep away invertebrates, but afterwards, macroalgae benefit from decreased grazing and increased light (Byrnes et al. 2011; Detmer et al. 2021). Furthermore, storms can aid the dispersal of fish eggs spawned on plants that get dislodged (Marliave 1976).
For some species groups, studies disagreed about the effects of a stressor. For example, most studies on bull kelp suggested adverse effects of warming, heat waves, and storms (e.g., reduced survival, growth, and reproduction) (Lind and Konar 2017; Muth et al. 2019; Burnett and Koehl 2022; Starko et al. 2022). However, temperature effects depend on whether the plants are near the warm or cold limits of their tolerated temperature range, and as an annual species, bull kelp is adapted to decreasing in abundance during the winter and regrowing during the spring (Hamilton et al. 2020). Indeed, some studies from other regions found that temperature did not affect kelp abundance in the same year, that long-term climate indices may be better predictors of kelp abundance (Pfister et al. 2018; Schroeder et al. 2020), and that storm waves did not drive local mortality (Duggins et al. 2001). Furthermore, the effects of a stressor sometimes differed between the species in a functional group. For example, some species of macroalgae cannot produce gametophytes at 18 °C or higher (e.g., Laminaria ephemera). Other species can complete their life cycle and recruit at 18 °C in the southern part of their range (e.g., Laminaria setchellii) (Muth et al. 2019). Such differences in the responses of species can cause local shifts in community composition and diversity (Starko et al. 2019). For example, during the 2014–2016 marine heatwave, species such as red turfy seaweeds replaced canopy-forming kelp forests at inshore sites in Barkley Sound (Starko et al. 2022).
The literature reported at least some adverse effects of climate stressors for all well-studied species groups. Still, net effects at the population or community level might be positive because of species interactions (e.g., reduced predation or competition), interactions with other stressors or other pathways, adaptation, or because related species benefit. The following paragraphs present three examples. Together, these examples illustrate that planning for ecological impacts of climate change must consider species-specific local knowledge and indirect effects, e.g., in comprehensive risk assessments (Harley 2011; Murray et al. 2016).
First, reported adverse effects of acidification on Mytilus trossulus mussels included limiting organisms to a smaller physical size in our study region (Sunday et al. 2011) and reduced recruitment and population size elsewhere in British Columbia (Brown et al. 2016). Adverse effects of warming included higher mortality of juveniles and reduced growth of adults (Jenewein and Gosselin 2013). In contrast, Whalen et al. (2023) found increased cover of Mytilus mussels (Mytilus californianus and M. trossulus, the former being dominant) following marine heatwaves and sea star wasting disease. Similar increases in M. trossulus after a heatwave, coincident with a reduced abundance of sea stars, have been reported from Alaska (Suryan et al. 2021; Weitzmann et al. 2021).
Second, warming and acidification adversely affect individual sea urchins (e.g., smaller body size and reduced gonad size; Sunday et al. 2011; Ebert et al. 2012). However, some sea urchin species can adapt to lower pH (Sunday et al. 2011; Pespeni et al. 2013; Evans et al. 2017), and in one field study, red sea urchin populations increased in a kelp forest after a heatwave (Starko et al. 2022).
Third, all life stages of Dungeness crabs will face increased risk from ocean acidification (Alin et al. 2023). At reduced pH, Dungeness crabs experience reduced development and survival (Miller et al. 2016), internal and external exoskeleton carapace dissolution, and reduced larval width (Bednaršek et al. 2020). At the same time, Dungeness crabs are cold-limited in the Northern Shelf Bioregion in British Columbia. They might initially benefit from warmer conditions due to larger suitable areas within marine protected areas (MPAs) and increased MPA interconnectedness (Friesen et al. 2021). Understanding the complex interplay of such processes at the ecosystem scale remains a key research challenge.
4. Discussion
Our pathways of effects model summarizes a diverse collection of local to regional-scale research on climate change effects on the coastal biota of western Vancouver Island and highlights the complexity of indirect effects and important knowledge gaps.
4.1. Climate change effects
Pathways of effects modelling highlighted the diversity of climate effects on the coastal biota of our study region. Documented effects ranged from changes in the body composition of individual organisms to higher-level effects like increased mortality rates and changed community composition. However, the literature had major gaps for some species groups, and some studies identified opposing effects for the well-studied groups (such as a detrimental effect on organisms in the lab but a beneficial effect on populations in the field). These might be due to indirect effects (e.g., trophic interactions) that are absent in lab studies, differences in study design (e.g., covarying environmental variables present in field studies but not in the lab), study sites and experiments with different initial conditions, locally adapted populations (see Section 3.3), or the limitations of this study (see Section 4.2).
Nevertheless, some effects had a consistent direction—e.g., increased risk of disease due to warming and reduced calcification due to acidification. Most pathways involved adverse effects like more disease (e.g., through harmful algal blooms), and reduced survival, growth, reproduction, and population size for many species groups. Hence, climate change threatens coastal ecosystems and human uses like fisheries on the West Coast of Vancouver Island, but its effects are manifold and complex. Understanding how ecosystem-scale changes might emerge from diverse pathways and species interactions remains a crucial challenge for future research.
Because our review focused on effects on coastal species, it does not comprehensively assess impacts that might concern people. For example, several studies from British Columbia and the U.S. West Coast found that warm ocean conditions and storms can intensify coastal Pseudo-nitzschia outbreaks, accumulating toxins in higher trophic levels (e.g., Dungeness crabs and anchovies). Although these toxins do not always negatively affect the species in which they accumulate, they pose a risk to commercial fisheries and seafood safety (McCabe et al. 2016; Ekstrom et al. 2020; Dudley et al. 2021; McIntyre et al. 2021).
4.2. Multiple stressors, multiple pathways, and pathways of effects models
Most species groups were affected by multiple stressors related to climate change. Yet coastal ecosystems are also subject to many non-climatic stressors (Stock et al. 2018), which can interact in complicated ways (Crain et al. 2008; Darling and Côté 2008; Côté et al. 2016). For example, warming can increase the risks associated with environmental contaminants (due to higher metabolic rates and, therefore, uptake of contaminants). Conversely, exposure to contaminants can reduce the ability of organisms to withstand climate-related stress (Noyes and Lema 2015). Local human activities might exacerbate or mitigate the effects of climate change (Gissi et al. 2021). Because ignoring interactions between stressors can lead to inefficient environmental protection and resource management (Brown et al. 2014), understanding multiple stressor effects is a research priority (Ryabinin et al. 2019; Borja et al. 2020). However, despite continued efforts and a sound understanding of species-level effects of temperature, there have been few generalizable results on the complex effects of climate change on coastal ecosystems. This is partly due to the complexity of stressor and species interactions and partly to difficulties integrating knowledge across scientific disciplines and research approaches (Harley et al. 2006; Côté et al. 2016; Halpern et al. 2019; Orr et al. 2020).
With this study, we have demonstrated how pathways of effects models can summarize scientific knowledge obtained with diverse research approaches and from intra-organism to community scales. Based on this broad body of research, our model showcases that even single stressor effects can be complex, as ecosystems can be affected by multiple pathways acting at different ecological, spatial, and temporal scales (Harley et al. 2006; Murray et al. 2014, 2020). Understanding and predicting the cumulative effects of stressors thus requires understanding the cumulative effects of multiple pathways.
4.3. Study scope and limitations
Several aspects of our data collection enabled the completion of this study but also limit the scope and nature of its conclusions. Our geographical hierarchy of searches (first seeking studies from the West Coast of Vancouver Island, then proceeding further and further afield) kept the number of studies manageable and regionally relevant. Our categorization of the diverse effects reported in the literature into general categories, and of species into functional groups, allowed for summarizing studies using different research approaches and reporting diverse outcome variables. However, five fundamental limitations follow from these methodological choices.
First, some identified pathways and directions of effects depend on the geographic location of the study reporting them. The West Coast of Vancouver Island and the neighbouring regions from which we drew some studies—from California to Alaska—span a wide range of environmental conditions. On Vancouver Island's complex coast, water temperature and other physical conditions vary locally (Mora-Sota et al. 2024). Some studies explicitly showed different effects across environmental gradients. For example, during the 2014–2016 marine heatwave, warm inshore areas in Barkley Sound (British Columbia) experienced extensive kelp loss, whereas populations in cooler waters on the outer coast persisted (Starko et al. 2022). Similarly, bull kelp declined in Northern California, yet populations on the cooler coast of Oregon were unresponsive or grew (Hamilton et al. 2020).
Second, our quantitative results, like the number of pathways in the model, depend on the chosen effect categories and species groups. For example, had we used individual species as endpoints, there would be many more pathways (because some pathways now ending with a group would split into several pathways for the constituent species).
Third, some species groups were broad and effects on some species might be negligible in others. For example, different rockfish species (here classified under “Other demersal reef fish”) respond differently to warming (Markel and Shurin 2020). The identified pathways, therefore, often reflect known climate effects on only a subset of the species in a group. Furthermore, the literature for some functional groups was biased towards a few species. For example, most research for predatory invertebrates studied the ochre sea star Pisaster ochraceus. If studies about all species in each group existed, they might have reported additional pathways or conflicting directions.
Fourth, while necessary to keep the number of studies manageable and focused on our study region, the geographical hierarchy of our literature search limits across-group comparisons. Consider the following example. A hypothetical Species Group 1 has no local or British Columbia studies and 15 studies from the U.S. West Coast. In contrast, Species Group 2 has five local studies and 50 studies from the U.S. West Coast. Following our hierarchical search protocol, the pathways of effects model would include all 15 U.S. studies for Group 1, but only the five local studies for Group 2. Thus, fewer included studies for Group 2 would not imply that it is less frequently studied globally. Considering literature from a broader geographical scope for this group might have revealed additional pathways. Furthermore, we used a different literature search method for the effects of acidification on giant kelp as our hierarchical procedure yielded either too few papers when searching locally or too many papers when searching globally. The results for this stressor and species group are thus not directly comparable to the others. To aid interpretation, we report the geographical focus of the studies and pathways throughout the text and figures.
Fifth, the included studies had diverse research designs. These ranged from lab experiments varying temperature to studies that inferred climate effects from monitoring data reflecting broad climatic cycles (with confounding changes in, e.g., upwelling and nutrient availability). The studies reflected diverse stressor intensities and durations of exposure, focused on different life stages, analysed diverse outcome metrics, and reflected different spatial and temporal scales. Furthermore, some older studies might not reflect the current methodological state of the art in their field. The evidence is thus not equally strong for all the reported pathways.
While these limitations rule out some kinds of conclusions, they do not affect our qualitative insights. For example, the conclusion that climate change effects on the coastal biota of the West Coast of Vancouver Island are manifold and complex does not depend on the exact number of pathways or differences in the geographical scope of the results across species groups. The supplementary materials document the geographical and literature biases for each species group.
4.4. Research gaps
We identified three research gaps. First, regional studies were sparse for many species groups. For others, even searches at broader geographical scales yielded few results. Consequently, many pathways were supported by a single study. Besides the local sea otter (Enhydra lutris, an iconic keystone species; Estes and Palmisano 1974) and small grazers, groups with few studies reporting climate change effects included kelp crabs, small heterotrophs, mesograzers, and many species of edible clams. Second, most literature focused on warming, with fewer studies on the effects of acidification and storms. For example, local studies about the effects of acidification were scarce and focused on mussels and sea urchins (but there were some studies from other places in British Columbia and many from the U.S. West Coast). Third, few studies investigated interactions between stressors. Because human impacts and ecological interactions can vary with the local context (e.g., Connell and Irving 2008; Hessing-Lewis et al. 2011), these research gaps are a barrier to regional, science-based marine management (Mach et al. 2015).
4.5. Future directions: towards pathways of effects databases
Our results emphasize two challenges for future research: (1) gaps in fundamental knowledge about climate effects on some species groups, and (2) a lack of synthesis of the many studies that already exist for others. To address these challenges, we suggest building standardized databases of known pathways of effects that centralize knowledge and allow comparisons across species and regions (Fig. 5). Such comparisons could be much simplified by ongoing efforts to unify the collection of data about the effects of specific stressors (e.g., Widdicombe et al. 2023). Below, we outline further research needs towards this goal and sketch potential applications in science and practice.
Fig. 5.

Because pathways of effects can differ between geographical locations, species, or populations, continued empirical research of climate change and multiple stressor effects at local to regional scales is fundamental. Furthermore, understanding stressor effects requires diverse research designs suitable for phenomena occurring at different ecological scales (Hodgson and Halpern 2019). With this study, we have demonstrated using pathways of effects models to concisely summarize scientific knowledge obtained with diverse methods and from intra-organism to community scales. Consequently, such models can be a helpful foundation for understanding human impacts and ecosystem changes in a region.
Ideally, pathways of effects models could be entered into queryable regional to global-scale databases in a unified format. Authors of relevant studies could add new studies to the database upon publication, increasing visibility to readers. Such databases would have many applications. For example, they would allow researchers to quickly summarize the state of knowledge about the effects of specific human activities or stressors on species, species groups, and geographical regions of interest. Researchers could also compare pathways across geographical regions, with the potential to yield hypotheses about what makes species and communities resilient to stressors. Finally, pathways of effects models can inform quantitative models that simulate population trajectories of endangered species under stress (Murray et al. 2021) or stressor effects across a food web (Stock et al. 2023). On the West Coast of Vancouver Island, we have laid a foundation for future simulations by using species groups from an existing quantitative food web model as endpoints (Gregr et al. 2020).
Beyond scientific applications, pathways of effects databases could provide a foundation for evidence- and ecosystem-based environmental management. For example, some environmental impact assessments rely on a rather coarse model of biological effects. A pathways of effects database allowing for regional queries could yield a concise summary of stressors caused by the respective activity (e.g., building and operating an offshore wind farm), known biological effects, and possible interactions with other stressors. This could provide a shared foundation for environmental impact assessments, helping proponents prepare them, but also enabling regulators to better evaluate them and ensure that potential environmental impacts are considered appropriately, which is arguably not the norm (Murray et al. 2018; Singh et al. 2019, 2020). A complementary search for other regions could reveal effects found for the same stressor or species group elsewhere and highlight local and global knowledge gaps.
4.6. Summary and conclusions
Climate change threatens marine ecosystems, as demonstrated for the coastal ecosystems and resources of the West Coast of Vancouver Island, with manifold and complex biological effects. Many species groups were affected by multiple climate-related stressors—often negatively—and even single stressors had varied effects across ecological scales. Some effects were region-specific, highlighting the need for local studies to better understand and manage these systems. Furthermore, some climate change effects were adverse at the organism level, yet net effects at the population level were positive because of species interactions, other pathways, or adaptation. We found conceptual pathways of effects models a helpful tool to summarize local studies across ecological scales. In the future, summarizing existing and new local studies into standardized pathways of effects databases could support important scientific and practical applications, such as ecosystem modelling, environmental impact assessments, and ecosystem-based management.
Acknowledgments
AS was supported by the Liber Ero Postdoctoral Program and a MEOPAR Postdoctoral Award. KMAC acknowledges support from his Canada Research Chair (CRC-2020-00183) and an NSERC Discovery Grant (RGPIN-2020-05032).
References
Adams N., MacFadyen A., Hickey B., Trainer V. 2006. The nearshore advection of a toxigenic Pseudo-nitzschia bloom and subsequent domoic acid contamination of intertidal bivalves. African Journal of Marine Science, 28(2): 271–276.
Alin S.R., Siedlecki S.A., Berger H., Feely R.A., Waddell J.E., Carter B.R., et al. 2023. Evaluating the evolving ocean acidification risk to dungeness crab. Oceanography, 36(2/3): 138–147.
Allen S.E., Wolfe M.A. 2013. Hindcast of the timing of the spring phytoplankton bloom in the Strait of Georgia, 1968–2010. Progress in Oceanography, 115, 6–13.
Bass A., Wernberg T., Thomsen M., Smale D. 2021. Another decade of marine climate change experiments: trends, progress and knowledge gaps. Frontiers in Marine Science, 8.
Bates A., Hilton B., Harley C. 2009. Effects of temperature, season and locality on wasting disease in the keystone predatory sea star Pisaster ochraceus. Diseases of Aquatic Organisms, 86, 245–251.
Bayne B.L., Bayne C.J., Carefoot T.C., Thompson R.J. 1976. The physiological ecology of Mytilus californianus Conrad. Oecologia, 22(3): 211–228.
Bednaršek N., Pelletier G., Ahmed A., Feely R.A. 2020. Chemical exposure due to anthropogenic ocean acidification increases risks for estuarine calcifiers in the Salish Sea: biogeochemical model scenarios. Frontiers in Marine Science, 7, 580.
Berger M.S., Emlet R.B. 2007. Heat-shock response of the upper intertidal barnacle Balanus glandula: thermal stress and acclimation. The Biological Bulletin, 212(3): 232–241.
Berry H.D., Mumford T.F., Christiaen B., Dowty P., Calloway M., Ferrier L., et al. 2021. Long-term changes in kelp forests in an inner basin of the Salish Sea. PLoS ONE, 16(2): e0229703.
Borja A., Andersen J.H., Arvanitidis C.D., Basset A., Buhl-Mortensen L., Carvalho S., et al. 2020. Past and future grand challenges in marine ecosystem ecology. Frontiers in Marine Science, 7(June).
Brierley A.S., Kingsford M.J. 2009. Impacts of climate change on marine organisms and ecosystems. Current Biology, 19(14): R602–R614.
Brown C.J., Saunders M.I., Possingham H.P., Richardson A.J. 2014. Interactions between global and local stressors of ecosystems determine management effectiveness in cumulative impact mapping. Diversity and Distributions, 20(5): 538–546.
Brown N.E.M., Therriault T.W., Harley C.D.G. 2016. Field-based experimental acidification alters fouling community structure and reduces diversity. Journal of Animal Ecology, 85(5): 1328–1339.
Brownscombe J., Smokorowski K. 2021. Review of Pathways of Effects (PoE) diagrams in support of FFHPP risk assessment (DFO Can. Sci. Advis. Sec. Res. Doc. 2021/079; p. iv+61). DFO.
Burnett N.P., Koehl M.A.R. 2022. Ecological biomechanics of damage to macroalgae. Frontiers in Plant Science, 13, 981904.
Burnham R.E., Meland K., Duffus D.A. 2017. First record of the marine mysid hippacanthomysis platypoda Murano & Chess, 1987 in coastal waters of British Columbia, Canada. Journal of Crustacean Biology, 37(4): 496–498.
Busch D.S., McElhany P. 2016. Estimates of the direct effect of seawater pH on the survival rate of species groups in the California current ecosystem. PLoS ONE, 11(8): e0160669.
Busch D.S., Maher M., Thibodeau P., McElhany P. 2014. Shell condition and survival of Puget Sound pteropods are impaired by ocean acidification conditions. PLoS ONE, 9(8): e105884.
Byrnes J.E., Reed D.C., Cardinale B.J., Cavanaugh K.C., Holbrook S.J., Schmitt R.J. 2011. Climate-driven increases in storm frequency simplify kelp forest food webs. Global Change Biology, 17(8): 2513–2524.
Carter H.A., Ceballos-Osuna L., Miller N.A., Stillman J.H. 2013. Impact of ocean acidification on metabolism and energetics during early life stages of the intertidal porcelain crab Petrolisthes cinctipes. Journal of Experimental Biology, 216(8): 1412–1422.
Castorani M.C.N., Bell T.W., Walter J.A.,Reuman D.C., Cavanaugh K.C., Sheppard L.W. 2022. Disturbance and nutrients synchronise kelp forests across scales through interacting Moran effects. Ecology Letters, 25(8): 1854–1868.
Cavallo M., Borja Á., Elliott M., Quintino V., Touza J. 2019. Impediments to achieving integrated marine management across borders: the case of the EU Marine Strategy Framework Directive. Marine Policy, 103, 68–73.
Chang A.S., Bertram M.A., Ivanochko T., Calvert S.E., Dallimore A., Thomson R.E. 2013. Annual record of particle fluxes, geochemistry and diatoms in Effingham Inlet, British Columbia, Canada, and the impact of the 1999 La Niña event. Marine Geology, 337, 20–34.
Checkley D.M., Dickson A.G., Takahashi M., Radich J.A., Eisenkolb N., Asch R. 2009. Elevated CO 2 enhances otolith growth in young fish. Science, 324(5935): 1683–1683.
Chen D.G., Irvine J.R. 2001. A semiparametric model to examine stock-recruitment relationships incorporating environmental data. Canadian Journal of Fisheries and Aquatic Sciences, 58(6): 1178–1186.
Chen Z., Shi J., Liu Q., Chen H., Li C. 2021. A persistent and intense marine heatwave in the Northeast Pacific during 2019–2020. Geophysical Research Letters, 48(13): e2021GL093239.
Connell S.D., Irving A.D. 2008. Integrating ecology with biogeography using landscape characteristics: a case study of subtidal habitat across continental Australia. Journal of Biogeography, 35(9): 1608–1621.
Cooley S., Schoeman D., Bopp L., Boyd P., Donner S., Ito S., et al. 2022. Oceans and coastal ecosystems and their services. In IPCC AR6 WGII. Cambridge University Press.
Cooper H.L., Potts D.C., Paytan A. 2016. Metabolic responses of the North Pacific krill, Euphausia pacifica, to short- and long-term pCO2 exposure. Marine Biology, 163(10): 207.
Cooper H.L., Potts D.C., Paytan A. 2017. Effects of elevated pCO2 on the survival, growth, and moulting of the Pacific krill species, Euphausia pacifica. ICES Journal of Marine Science, 74(4): 1005–1012.
Côté I.M., Darling E.S., Brown C.J. 2016. Interactions among ecosystem stressors and their importance in conservation. Proceedings of the Royal Society B: Biological Sciences, 283(1824): 20152592.
Crain C.M., Kroeker K., Halpern B.S. 2008. Interactive and cumulative effects of multiple human stressors in marine systems. Ecology Letters, 11(12): 1304–1315.
Crawford W.R., Thomson R.E. 1991. Physical oceanography of the western Canadian continental shelf. Continental Shelf Research, 11(8–10): 669–683.
Crim R.N., Sunday J.M., Harley C.D.G. 2011. Elevated seawater CO2 concentrations impair larval development and reduce larval survival in endangered northern abalone (Haliotis kamtschatkana). Journal of Experimental Marine Biology and Ecology, 400(1–2): 272–277.
Crowder L., Norse E. 2008. Essential ecological insights for marine ecosystem-based management and marine spatial planning. Marine Policy, 32(5): 772–778.
Darling E.S., Côté I.M. 2008. Quantifying the evidence for ecological synergies. Ecology Letters, 11(12): 1278–1286.
Detmer A.R., Miller R.J., Reed D.C., Bell T.W., Stier A.C., Moeller H.V. 2021. Variation in disturbance to a foundation species structures the dynamics of a benthic reef community. Ecology, 102(5): e03304.
Deysher E., Dean T.A. 1986. In situ recruitment of sporophytes of the giant kelp, Macrocystis pyrijkra (L.) C.A. Agardh: effects of physical factors. 23.
Di Lorenzo E., Mantua N. 2016. Multi-year persistence of the 2014/15 North Pacific marine heatwave. Nature Climate Change, 6(11): 1042–1047.
Dudas S., Dower J. 2006. Reproductive ecology and dispersal potential of varnish clam Nuttallia obscurata, a recent invader in the Northeast Pacific Ocean. Marine Ecology Progress Series, 320, 195–205.
Dudley P.N., Rogers T.L., Morales M.M., Stoltz A.D., Sheridan C.J., Beulke A.K., et al. 2021. A more comprehensive climate vulnerability assessment framework for fisheries social-ecological systems. Frontiers in Marine Science, 8, 678099.
Duggins D., Eckman J., Siddon C., Klinger T. 2001. Interactive roles of mesograzers and current flow in survival of kelps. Marine Ecology Progress Series, 223, 143–155.
Ebert T., Hernández J., Russell M. 2012. Ocean conditions and bottom-up modifications of gonad development in the sea urchin Strongylocentrotus purpuratus over space and time. Marine Ecology Progress Series, 467, 147–166.
Eckert G.L., Engle J.M., Kushner D.J. 2002. Sea star disease and population declines AT the channel islands. 4.
Ekstrom J.A., Moore S.K., Klinger T. 2020. Examining harmful algal blooms through a disaster risk management lens: a case study of the 2015 U.S. West Coast domoic acid event. In Harmful Algae. Vol. 94. p. 101740.
El-Sabaawi R.W., Trudel M., Mackas D.L., Dower J.F., Mazumder A. 2012. Interannual variability in bottom-up processes in the upstream range of the California Current system: an isotopic approach. Progress in Oceanography, 106, 16–27.
El-Sabaawi R., Trudel M., Mazumder A. 2013. Zooplankton stable isotopes as integrators of bottom-up variability in coastal margins: a case study from the Strait of Georgia and adjacent coastal regions. Progress in Oceanography, 115, 76–89.
Epelbaum A., Herborg L.M., Therriault T.W., Pearce C.M. 2009. Temperature and salinity effects on growth, survival, reproduction, and potential distribution of two non-indigenous botryllid ascidians in British Columbia. Journal of Experimental Marine Biology and Ecology, 369(1): 43–52.
Estes J.A., Palmisano J.F. 1974. Sea otters: their role in structuring nearshore communities. Science, 185(4156): 1058–1060.
Evans R., English P.A., Gauthier S., Robinson C.L.K. 2023. Quantifying the effects of extreme events and oceanographic variability on the spatiotemporal biomass and distribution of two key euphausiid prey species. Frontiers in Marine Science, 10, 1031485.
Evans T.G., Pespeni M.H., Hofmann G.E., Palumbi S.R., Sanford E. 2017. Transcriptomic responses to seawater acidification among sea urchin populations inhabiting a natural pH mosaic. Molecular Ecology, 26(8): 2257–2275.
Fernández P.A., Navarro J.M., Camus C., Torres R., Buschmann A.H. 2021. Effect of environmental history on the habitat-forming kelp Macrocystis pyrifera responses to ocean acidification and warming: a physiological and molecular approach. Scientific Reports, 11(1): 2510.
Fieber A.M., Bourdeau P.E. 2021. Elevated pCO2 reinforces preference among intertidal algae in both a specialist and generalist herbivore. Marine Pollution Bulletin, 168, 112377.
Foreman R.E. n.d. Studies on nereocystis growth in British Columbia, Canada. 8.
Frank P.W. 1975. Latitudinal variation in the life history features of the black Turban snail Tegula funebralis (Prosobranchia: Trochidae). 12.
Friesen S.K., Rubidge E., Martone R., Hunter K.L., Peña M.A., Ban N.C. 2021. Effects of changing ocean temperatures on ecological connectivity among marine protected areas in northern British Columbia. Ocean & Coastal Management, 211, 105776.
Gaitán-Espitia J.D., Hancock J.R., Padilla-Gamiño J.L., Rivest E.B., Blanchette C.A., Reed D.C., Hofmann G.E. 2014. Interactive effects of elevated temperature and pCO2 on early-life-history stages of the giant kelp Macrocystis pyrifera. Journal of Experimental Marine Biology and Ecology, 457, 51–58.
Garza C., Robles C. 2010. Effects of brackish water incursions and diel phasing of tides on vertical excursions of the keystone predator Pisaster ochraceus. Marine Biology, 157(3): 673–682.
Gaylord B., Hill T.M., Sanford E., Lenz E.A., Jacobs L.A., Sato K.N., et al. 2011. Functional impacts of ocean acidification in an ecologically critical foundation species. Journal of Experimental Biology, 214(15): 2586–2594.
Gissi E., Manea E., Mazaris A.D., Fraschetti S., Almpanidou V., Bevilacqua S., et al. 2021. A review of the combined effects of climate change and other local human stressors on the marine environment. Science of the Total Environment, 755(September 2020): 142564.
Godefroid M., Boldt J.L., Thorson J.T., Forrest R., Gauthier S., Flostrand L., et al. 2019. Spatio-temporal models provide new insights on the biotic and abiotic drivers shaping Pacific Herring (Clupea pallasi) distribution. Progress in Oceanography, 178, 102198.
Gooding R.A., Harley C.D.G., Tang E. 2009. Elevated water temperature and carbon dioxide concentration increase the growth of a keystone echinoderm. Proceedings of the National Academy of Sciences, 106(23): 9316–9321.
Government of Canada. 2012. Pathways of Effects National Guidelines. Fisheries and Oceans Canada. p. 32.
Graham M., Harrold C., Lisin S., Light K., Watanabe J., Foster M. 1997. Population dynamics of giant kelp Macrocystis pyrifera along a wave exposure gradient. Marine Ecology Progress Series, 148, 269–279.
Gray M., Langdon C., Waldbusser G., Hales B., Kramer S. 2017. Mechanistic understanding of ocean acidification impacts on larval feeding physiology and energy budgets of the mussel Mytilus californianus. Marine Ecology Progress Series, 563, 81–94.
Gregr E.J., Christensen V., Nichol L., Martone R.G., Markel R.W., Watson J.C., et al. 2020. Cascading social–ecological costs and benefits triggered by a recovering keystone predator. Science, 368(6496): 1243–1247.
Gurr S.J., Vadopalas B., Roberts S.B., Putnam H.M. 2020. Metabolic recovery and compensatory shell growth of juvenile Pacific geoduck Panopea generosa following short-term exposure to acidified seawater. Conservation Physiology, 8(1): coaa024.
Gurr S.J., Trigg S.A., Vadopalas B., Roberts S.B., Putnam H.M. 2021. Repeat exposure to hypercapnic seawater modifies growth and oxidative status in a tolerant burrowing clam. Journal of Experimental Biology, 224(13): jeb233932.
Haigh R., Taylor F., Sutherland T. 1992. Phytoplankton ecology of Sechelt Inlet, a fjord system on the British Columbia coast. I. General features of the nano- and microplankton. Marine Ecology Progress Series, 89, 117–134.
Haigh R., Ianson D., Holt C.A., Neate H.E., Edwards A.M. 2015. Effects of ocean acidification on temperate coastal marine ecosystems and fisheries in the Northeast Pacific. PLoS ONE, 10(2): e0117533.
Halpern B.S., Hodgson E.E., Essington T.E. 2019. Moving beyond silos in cumulative effects assessment. Frontiers in Ecology and Evolution, 7, 211.
Hamilton S.L., Bell T.W., Watson J.R., Grorud-Colvert K.A., Menge B.A. 2020. Remote sensing: generation of long-term kelp bed data sets for evaluation of impacts of climatic variation. Ecology, 101(7): e03031.
Hamilton T.J., Holcombe A., Tresguerres M. 2014. CO 2-induced ocean acidification increases anxiety in Rockfish via alteration of GABAA receptor functioning. Proceedings of the Royal Society B: Biological Sciences, 281(1775): 20132509.
Hannah L., Thornborough K., Clarke Murray C., Nelson J., Locke A., Mortimor J., Lawson J. 2020. Pathways of effects conceptual models for marine commercial shipping in Canada: biological and ecological effects. Canadian Science Advisory Secretariat. p. 212.
Harley C.D.G. 2011. Climate change, keystone predation, and biodiversity loss. Science, 334(6059): 1124–1127.
Harley C.D.G., Hughes A.R., Hultgren K.M., Miner B.G., Sorte C.J.B., Thornber C.S., et al. 2006. The impacts of climate change in coastal marine systems. Ecology Letters, 9(2): 228–241.
Harris S.L., Varela D.E., Whitney F.W., Harrison P.J. 2009. Nutrient and phytoplankton dynamics off the west coast of Vancouver Island during the 1997/98 ENSO event. Deep Sea Research Part II: Topical Studies in Oceanography, 56(24): 2487–2502.
Hay M.B., Dallimore A., Thomson R.E., Calvert S.E., Pienitz R. 2007. Siliceous microfossil record of late holocene oceanography and climate along the west coast of Vancouver Island, British Columbia (Canada). Quaternary Research, 67(1): 33–49.
Helmuth B., Broitman B.R., Blanchette C.A., Gilman S., Halpin P., Harley C.D.G., et al. 2006. Mosaic patterns of thermal stress in the Rocky Intertidal Zone: implications for climate change. Ecological Monographs, 76(4): 461–479.
Hesketh A.V., Harley C.D.G. 2023. Extreme heatwave drives topography-dependent patterns of mortality in a bed-forming intertidal barnacle, with implications for associated community structure. Global Change Biology, 29(1): 165–178.
Hessing-Lewis M.L., Hacker S.D., Menge B.A., Rumrill S.S. 2011. Context-dependent eelgrass–macroalgae interactions along an estuarine gradient in the Pacific Northwest, USA. Estuaries and Coasts, 34(6): 1169–1181.
Hettinger A., Sanford E., Hill T.M., Russell A.D., Sato K.N.S., Hoey J., et al. 2012. Persistent carry-over effects of planktonic exposure to ocean acidification in the Olympia oyster. Ecology, 93(12): 2758–2768.
Hodgson E.E., Halpern B.S. 2019. Investigating cumulative effects across ecological scales. Conservation Biology, 33(1): 22–32.
Hoegh-Guldberg O., Bruno J.F. 2010. The impact of climate change on the world's marine ecosystems. Science, 328(5985): 1523–1528.
Holbrook S.J., Schmitt R.J., Stephens J.S. Jr. 1997. Changes in an assemblage of temperate reef fishes associated with a climate shift. Ecological Applications, 7(4): 1299–1310.
Hollarsmith J.A., Buschmann A.H., Camus C., Grosholz E.D. 2020. Varying reproductive success under ocean warming and acidification across giant kelp (Macrocystis pyrifera) populations. Journal of Experimental Marine Biology and Ecology, 522, 151247.
Hollarsmith J.A., Andrews K., Naar N., Starko S., Calloway M., Obaza A., et al. 2022. Toward a conceptual framework for managing and conserving marine habitats: a case study of kelp forests in the Salish Sea. Ecology and Evolution 12(1): e8510.
Ito S., Rose K.A., Megrey B.A., Schweigert J., Hay D., Werner F.E., Aita M.N. 2015. Geographic variation in Pacific herring growth in response to regime shifts in the North Pacific Ocean. Progress in Oceanography, 138, 331–347.
Iwabuchi B.L., Gosselin L.A. 2019. Long-term trends and regional variability in extreme temperature and salinity conditions experienced by coastal marine organisms on Vancouver Island, Canada.Bulletin of Marine Science, 95(3): 337–354.
Jellison B.M., Ninokawa A.T., Hill T.M., Sanford E., Gaylord B. 2016. Ocean acidification alters the response of intertidal snails to a key sea star predator. Proceedings of the Royal Society B: Biological Sciences, 283(1833): 20160890.
Jenewein B., Gosselin L. 2013. Ontogenetic shift in stress tolerance thresholds of Mytilus trossulus: effects of desiccation and heat on juvenile mortality. Marine Ecology Progress Series, 481, 147–159.
Johnson K.A., Yoklavich M.M., Cailliet G.M. 2001. Recruitment of three species of Juvenile rockfish (S€BAST€S SPP.) on soft benthic habitat in Monterey Bay, California. 42.
Kay S.W.C., Gehman A.-L.M., Harley C.D.G. 2019. Reciprocal abundance shifts of the intertidal sea stars, Evasterias troschelii and Pisaster ochraceus, following sea star wasting disease. Proceedings of the Royal Society B: Biological Sciences, 286(1901): 20182766.
Kelly M.W., Padilla-Gamiño J.L., Hofmann G.E. 2013. Natural variation and the capacity to adapt to ocean acidification in the keystone sea urchin Strongylocentrotus purpuratus. Global Change Biology, 19(8): 2536–2546.
Konar B., Estes J.A. 2003. The stability of boundary regions between Kelp Beds and deforested areas. Ecology, 84(1): 174–185.
Kordas R., Harley C. 2016. Demographic responses of coexisting species to in situ warming. Marine Ecology Progress Series, 546, 147–161.
Kroeker K.J., Powell C., Donham E.M. 2021. Windows of vulnerability: seasonal mismatches in exposure and resource identity determine ocean acidification's effect on a primary consumer at high latitude. Global Change Biology, 27(5): 1042–1051.
Lam L.S., Basnett B.L., Haltuch M.A., Cope J., Andrews K., Nichols K.M., et al. 2021. Geographic variability in lingcod ophiodon elongatus life history and demography along the US West Coast: oceanographic drivers and management implications. Marine Ecology Progress Series, 670, 203–222.
Leal P.P., Hurd C.L., Fernández P.A., Roleda M.Y. 2017. Ocean acidification and kelp development: reduced pH has no negative effects on meiospore germination and gametophyte development of Macrocystis pyrifera and Undaria pinnatifida. Journal of Phycology, 53(3): 557–566.
Lester S.E., Tobin E.D., Behrens M.D. 2007. Disease dynamics and the potential role of thermal stress in the sea urchin, Strongylocentrotus purpuratus. Canadian Journal of Fisheries and Aquatic Sciences, 64(2): 314–323.
Leys S., Wilson K., Holeton C., Reiswig H., Austin W., Tunnicliffe V. 2004. Patterns of glass sponge (Porifera, Hexactinellida) distribution in coastal waters of British Columbia, Canada. Marine Ecology Progress Series, 283, 133–149.
Lind A.C., Konar B. 2017. Effects of abiotic stressors on kelp early life-history stages. ALGAE, 32(3): 223–233.
Lochead J., Gillespie D., Hand C. 2012. Storm-induced anastrophic burial of the Pacific geoduck (Panopea generosa) on the west coast of Vancouver Island. Journal of Shellfish Research, 31(4): 959–967.
Long W.C., Swiney K.M., Foy R.J. 2013. Effects of ocean acidification on the embryos and larvae of red king crab, Paralithodes camtschaticus. Marine Pollution Bulletin, 69(1–2): 38–47.
Mach M.E., Martone R.G., Chan K.M.A. 2015. Human impacts and ecosystem services: insufficient research for trade-off evaluation. Ecosystem Services, 16, 112–120.
Mackas D.l., Thomson R.E., Galbraith M. 2001. Changes in the zooplankton community of the British Columbia continental margin, 1985–1999, and their covariation with oceanographic conditions. Canadian Journal of Fisheries & Aquatic Sciences, 58(4): 685.
Mackas D.L., Peterson W.T., Zamon J.E. 2004. Comparisons of interannual biomass anomalies of zooplankton communities along the continental margins of British Columbia and Oregon. Deep Sea Research Part II: Topical Studies in Oceanography, 51(6–9): 875–896.
Markel R.W., Shurin J.B. 2020. Contrasting effects of coastal upwelling on growth and recruitment of nearshore Pacific rockfishes (genus Sebastes). Canadian Journal of Fisheries & Aquatic Sciences, 77(6): 950–962.
Markel R., Lotterhos K., Robinson C. 2017. Temporal variability in the environmental and geographic predictors of spatial-recruitment in nearshore rockfishes. Marine Ecology Progress Series, 574, 97–111.
Marliave J.B. 1976. A theory of storm-induced drift dispersal of the gasterosteid fish Aulorhynchus flavidus. Copeia, 1976(4): 794–796.
Marshall R., McKinley R.S., Pearce C.M. 2012. Effect of temperature on gonad development of the Pacific geoduck clam (Panopea generosa Gould, 1850). Aquaculture, 338–341, 264–273.
McCabe R.M., Hickey B.M., Kudela R.M., Lefebvre K.A., Adams N.G., Bill B.D., et al. 2016. An unprecedented coastwide toxic algal bloom linked to anomalous ocean conditions. Geophysical Research Letters, 43(19): 10,366–10,376.
McIntyre L., Miller A., Kosatsky T. 2021. Changing trends in paralytic shellfish poisonings reflect increasing sea surface temperatures and practices of Indigenous and recreational harvesters in British Columbia, Canada. Marine Drugs, 19(10): 568.
McLaskey A.K., Keister J.E. 2021. An integrated field-laboratory investigation of the effects of low oxygen and pH on North Pacific krill (Euphausia pacifica). Marine Biology, 168(4): 43.
Miller J.J., Maher M., Bohaboy E., Friedman C.S., McElhany P. 2016. Exposure to low pH reduces survival and delays development in early life stages of Dungeness crab (Cancer magister). Marine Biology, 163(5): 118.
Milligan K.L.D., DeWreede R.E. 2000. Variations in holdfast attachment mechanics with developmental stage, substratum-type, season, and wave-exposure for the intertidal kelp species Hedophyllum sessile (C. Agardh) Setchell. Journal of Experimental Marine Biology and Ecology, 254(2): 189–209.
Mora-Soto A., Schroeder S., Gendall L., Wachmann A., Narayan G.R., Read S., et al. 2024. Kelp dynamics and environmental drivers in the southern Salish Sea, British Columbia, Canada. Frontiers in Marine Science, 11, 1323448.
Murray C.C., Mach M.E., Martone R.G. 2014. Cumulative effects in marine ecosystems: scientific perspectives on its challenges and solutions. World Wildlife Federation Canada and Center for Ocean Solutions.
Murray C.C., Agbayani S., Ban N.C. 2015. Cumulative effects of planned industrial development and climate change on marine ecosystems. Global Ecology and Conservation, 4, 110–116.
Murray C.C., Mach M.E., Martone R.G., Singh G.G., O M., Chan K.M.A. 2016. Supporting risk assessment: accounting for indirect risk to ecosystem components. PLoS ONE, 11(9): e0162932.
Murray C.C., Wong J., Singh G.G., Mach M., Lerner J., Ranieri B., et al. 2018. The insignificance of thresholds in environmental impact assessment: an illustrative case study in Canada. Environmental Management, 61(6): 1062–1071.
Murray C.C., Hannah L.C., Doniol-Valcroze T., Wright B., Stredulinsky E., Locke A., Lacy R. 2019. Cumulative effects assessment for Northern and Southern resident killer whale populations in the Northeast Pacific. Canadian Science Advisory Secretariat. p. 88.
Murray C.C., Hannah L.C., Locke A. 2020. A review of cumulative effects research and assessment in Fisheries and Oceans Canada (3357; Can. Tech. Rep. Fish. Aquat. Sci., p. vii + 51). Fisheries and Oceans Canada.
Murray C.C., Hannah L.C., Doniol-valcroze T., Wright B.M., Stredulinsky E.H., Nelson J.C., et al. 2021. A cumulative effects model for population trajectories of resident killer whales in the Northeast Pacific. Biological Conservation, 257, 109124.
Muth A.F., Graham M.H., Lane C.E., Harley C.D.G. 2019. Recruitment tolerance to increased temperature present across multiple kelp clades. Ecology, 100(3): e02594.
Noakes D.J., Campbell A. 1992. Use of geoduck clams to indicate changes in the marine environment of Ladysmith Harbour, British Columbia. Environmetrics, 3(1): 81–97.
Noyes P.D., Lema S.C. 2015. Forecasting the impacts of chemical pollution and climate change interactions on the health of wildlife. Current Zoology, 61(4): 669–689.
Orr J.A., Vinebrooke R.D., Jackson M.C., Kroeker K.J., Kordas R.L., Mantyka-pringle C., et al. 2020. Towards a unified study of multiple stressors: divisions and common goals across research disciplines. Proceedings of the Royal Society B: Biological Sciences, 287, 20200421.
Osovitz C.J., Hofmann G.E. 2005. Thermal history-dependent expression of the hsp70 gene in purple sea urchins: biogeographic patterns and the effect of temperature acclimation. Journal of Experimental Marine Biology and Ecology, 327(2): 134–143.
Padilla-Gamiño J.L., Kelly M.W., Evans T.G., Hofmann G.E. 2013. Temperature and CO2 additively regulate physiology, morphology and genomic responses of larval sea urchins, Strongylocentrotus purpuratus. Proceedings of the Royal Society B: Biological Sciences, 280(1759): 20130155.
Patterson R.T., Prokoph A., Kumar A., Chang A.S., Roe H.M. 2005. Late Holocene variability in pelagic fish scales and dinoflagellate cysts along the west coast of Vancouver Island, NE Pacific Ocean. Marine Micropaleontology, 55(3–4): 183–204.
Pespeni M.H., Chan F., Menge B.A., Palumbi S.R. 2013. Signs of adaptation to local pH conditions across an environmental mosaic in the California current ecosystem. Integrative and Comparative Biology, 53(5): 857–870.
Peterson W.T., Keister J.E., Feinberg L.R. 2002. The effects of the 1997–99 El Niño/La Niña events on hydrography and zooplankton off the central Oregon coast. Progress in Oceanography, 54(1): 381–398.
Pfister C.A., Berry H.D., Mumford T. 2018. The dynamics of Kelp Forests in the Northeast Pacific Ocean and the relationship with environmental drivers. Journal of Ecology, 106(4): 1520–1533.
Philip S.Y., Kew S.F., van Oldenborgh G.J., Anslow F.S., Seneviratne S.I., Vautard R., et al. 2022. Rapid attribution analysis of the extraordinary heat wave on the Pacific coast of the US and Canada in June 2021. Earth System Dynamics, 13(4): 1689–1713.
Pickard D., Porter M., Olson E., Connors B., Kellock K., Jones E. 2015. Skeena River Estuary Assessment: Technical Report. Pacific Salmon Foundation, Vancouver, BC.
Pincebourde S., Sanford E., Helmuth B. 2008. Body temperature during low tide alters the feeding performance of a top intertidal predator. Limnology and Oceanography, 53(4): 1562–1573.
Place S., O'Donnell M., Hofmann G. 2008. Gene expression in the intertidal mussel Mytilus californianus: physiological response to environmental factors on a biogeographic scale. Marine Ecology Progress Series, 356, 1–14.
Poloczanska E.S., Brown C.J., Sydeman W.J., Kiessling W., Schoeman D.S., Moore P.J., et al. 2013. Global imprint of climate change on marine life. Nature Climate Change, 3(10): 919–925.
Raymond W.W., Barber J.S., Dethier M.N., Hayford H.A., Harley C.D.G., King T.L., et al. 2022. Assessment of the impacts of an unprecedented heatwave on intertidal shellfish of the Salish Sea. Ecology, 103(10):.
Riebesell U., Zondervan I., Zeebe R.E. 2000. Reduced calci®cation of marine plankton in response to increased atmospheric CO2. 407, 4.
Rogers-Bennett L. 2007. Is climate change contributing to range reductions and localized extinctions in northern (Haliotis kamtschatkana) and flat (Haliotis walallensis) abalones? Bulletin of Marine Science, 81(2): 15.
Roleda M.Y., Morris J.N., McGraw C.M., Hurd C.L. 2012. Ocean acidification and seaweed reproduction: increased CO2 ameliorates the negative effect of lowered pH on meiospore germination in the giant kelp Macrocystis pyrifera (Laminariales, Phaeophyceae). Global Change Biology, 18(3): 854–864.
Ryabinin V., Barbière J., Haugan P., Kullenberg G., Smith N., McLean C., et al. 2019. The UN decade of ocean science for sustainable development. Frontiers in Marine Science, 6(JUL):.
Sagarin R.D., Somero G.N. 2006. Complex patterns of expression of heat-shock protein 70 across the southern biogeographical ranges of the intertidal mussel Mytilus californianus and snail Nucella ostrina. Journal of Biogeography, 33(4): 622–630.
Sanford E. 1999. Regulation of keystone predation by small changes in ocean temperature. Science, 283(5410): 2095–2097.
Sanford E., Gaylord B., Hettinger A., Lenz E.A., Meyer K., Hill T.M. 2014. Ocean acidification increases the vulnerability of native oysters to predation by invasive snails. Proceedings of the Royal Society B: Biological Sciences, 281(1778): 20132681.
Schroeder S.B., Boyer L., Juanes F., Costa M. 2020. Spatial and temporal persistence of nearshore kelp beds on the west coast of British Columbia, Canada using satellite remote sensing. Remote Sensing in Ecology and Conservation, 6(3): 327–343.
Seymour R.J., Tegner M.J., Dayton P.K., Parnell P.E. 1989. Storm wave induced mortality of giant kelp, Macrocystis pyrifera, in Southern California. Estuarine, Coastal and Shelf Science, 28(3): 277–292.
Singh G.G., Lerner J., Clarke Murray C., Wong J., Mach M., Ranieri B., et al. 2019. Response to critique of “the Insignificance of thresholds in environmental Impact Assessment: an illustrative case Study in Canada. Environmental Management, 64(2): 133–137.
Singh G.G., Lerner J., Mach M., Murray C.C., Ranieri B., St-Laurent G.P., et al. 2020. Scientific shortcomings in environmental impact statements internationally. People and Nature, 2(2): 369–379.
Smith J.A.C., Galbraith M., Young K., Perry R.I., Sastri A., Nelson R.J. 2021. Acartia arbruta (previously A. tonsa) in British Columbia: a bioindicator of climate-driven ecosystem variability in the northeast Pacific Ocean. Journal of Plankton Research, 43(4): 546–564.
Starko S., Bailey L.A., Creviston E., James K.A., Warren A., Brophy M.K., et al. 2019. Environmental heterogeneity mediates scale-dependent declines in kelp diversity on intertidal rocky shores. PLoS ONE, 14(3): e0213191.
Starko S., Neufeld C.J., Gendall L., Timmer B., Campbell L., Yakimishyn J., et al. 2022. Microclimate predicts kelp forest extinction in the face of direct and indirect marine heatwave effects. Ecological Applications, 32(7): e2673.
Stevenson A., Archer S.K., Schultz J.A., Dunham A., Marliave J.B., Martone P., Harley C.D.G. 2020. Warming and acidification threaten glass sponge aphrocallistes vastus pumping and reef formation. Scientific Reports, 10(1): 8176.
Stock A., Crowder L.B., Halpern B.S., Micheli F. 2018. Uncertainty analysis and robust areas of high and low modeled human impact on the global oceans. Conservation Biology, 32(6): 1368–1379.
Stock A., Murray C.C., Gregr E.J., Christensen V., Steenbeek J., Woodburn E., et al. 2023. Exploring cumulative human impacts with Ecopath, Ecosim, and Ecospace: research designs, modeling techniques, and future opportunities. Science of The Total Environment, 869(161719).
Sulkin S.D., McKeen G.L. 1996. Larval development of the crabCancer magister in temperature regimes simulating outer-coast and inland-water habitats. Marine Biology, 127(2): 235–240.
Sunday J.M., Crim R.N., Harley C.D.G., Hart M.W. 2011. Quantifying rates of evolutionary adaptation in response to ocean acidification. PLoS ONE, 6(8): e22881.
Supratya V.P., Coleman L.J.M., Martone P.T. 2020. Elevated temperature affects phenotypic plasticity in the bull kelp (Nereocystis luetkeana, Phaeophyceae). Journal of Phycology, 56(6): 1534–1541.
Suryan R.M., Arimitsu M.L., Coletti H.A., Hopcroft R.R., Lindeberg M.R., Barbeaux S.J., et al. 2021. Ecosystem response persists after a prolonged marine heatwave. Scientific reports, 11(1): 6235.
Sussmann A.V., DeWreede R.E. 2005. Survival of the endophytic sporophyte of Acrosiphonia (Codiolales, Chlorophyta). Journal of the Marine Biological Association of the United Kingdom, 85(1): 49–58.
Sussmann A.V., Scrosati R., DeWreede R.E. 2005. Seasonal synchrony of a green algal endophyte, Acrosiphonia (Codiolales), with its red algal hosts, Mastocarpus and Mazzaella (Gigartinales). Phycologia, 44(1): 129–132.
Szathmary P., Helmuth B., Wethey D. 2009. Climate change in the rocky intertidal zone: predicting and measuring the body temperature of a keystone predator. Marine Ecology Progress Series, 374, 43–56.
Tanasichuk R.W. 1997. Influence of biomass and ocean climate on the growth of Pacific herring (Clupea pallasi) from the southwest coast of Vancouver Island. Canadian Journal of Fisheries and Aquatic Sciences, 54(12): 2782–2788.
Timmins-Schiffman E., Guzmán J.M., Elliott Thompson R., Vadopalas B., Eudeline B., Roberts S.B. 2020. Dynamic response in the larval geoduck (Panopea generosa) proteome to elevated p CO2. Ecology and Evolution, 10(1): 185–197.
Timothy Patterson R., Chang A.S., Prokoph A., Roe H.M., Swindles G.T. 2013. Influence of the Pacific Decadal Oscillation, El Niño-Southern Oscillation and solar forcing on climate and primary productivity changes in the northeast Pacific. Quaternary International, 310, 124–139.
Tolimieri N., Shelton A.O., Samhouri J.F., Harvey C.J., Feist B.E., Williams G.D., et al. 2023. Changes in kelp forest communities off Washington, USA, during and after the 2014–2016 marine heatwave and sea star wasting syndrome. Marine Ecology Progress Series, 703, 47–66.
Trainer V.L., Hickey B.M., Horner R.A. 2002. Biological and physical dynamics of domoic acid production off the Washington coast. Limnology and Oceanography, 47(5): 1438–1446.
Trigg S.A., McElhany P., Maher M., Perez D., Busch D.S., Nichols K.M. 2019. Uncovering mechanisms of global ocean change effects on the Dungeness crab (Cancer magister) through metabolomics analysis. Scientific Reports, 9(1): 10717.
Turley B.D., Rykaczewski R.R. 2019. Influence of wind events on larval fish mortality rates in the southern California Current Ecosystem. Canadian Journal of Fisheries and Aquatic Sciences, 76(12): 2418–2432.
Venello T.A., Sastri A.R., Suchy K.D., Galbraith M.D., Dower J.F. 2021. Drivers of variation in crustacean zooplankton production rates differ across regions off the west coast of Vancouver Island and in the subarctic NE Pacific. ICES Journal of Marine Science, 79, 741.
Villalobos C., Love B.A., Olson M.B. 2020. Ocean acidification and Ocean warming effects on Pacific herring (Clupea pallasi) early life stages. Frontiers in Marine Science, 7: 597899.
Ware D.M., Thomson R.E. 2005. Bottom-up ecosystem trophic dynamics determine fish production in the Northeast Pacific. Science, 308(5726): 1280–1284.
Weitzman B., Konar B., Iken K., Coletti H., Monson D., Suryan R., et al. 2021. Changes in rocky intertidal community structure during a marine heatwave in the northern Gulf of Alaska. Frontiers in Marine Science, 8: 556820.
Whalen M.A., Starko S., Lindstrom S.C., Martone P.T. 2023. Heatwave restructures marine intertidal communities across a stress gradient. Ecology, 104(5): e4027.
Widdicombe S., Isensee K., Artioli Y., Gaitán-Espitia J.D., Hauri C., Newton J.A., et al. 2023. Unifying biological field observations to detect and compare ocean acidification impacts across marine species and ecosystems: what to monitor and why. Ocean Science, 19(1): 101–119.
Wiebe J.P. 1968. The effects of temperature and daylength on the reproductive physiology of the viviparous seaperch, cymatogaster aggregata Gibbons. Canadian Journal of Zoology, 46(6): 1207–1219.
Wilson A.B. 2009. Fecundity selection predicts Bergmann's rule in syngnathid fishes. Molecular Ecology, 18(6): 1263–1272.
Xu Y., Fu C., Peña A., Hourston R., Thomson R., Robinson C., et al. 2019. Variability of Pacific herring (Clupea pallasii) spawn abundance under climate change off the West Coast of Canada over the past six decades. Journal of Marine Systems, 200: 103229.
Xuereb A., Kimber C.M., Curtis J.M.R., Bernatchez L., Fortin M.-J. 2018. Putatively adaptive genetic variation in the giant California sea cucumber (Parastichopus californicus) as revealed by environmental association analysis of restriction-site associated DNA sequencing data. Molecular Ecology, 27(24): 5035–5048.
Xuereb A., D'Aloia C.C., Andrello M., Bernatchez L., Fortin M.-J. 2021. Incorporating putatively neutral and adaptive genomic data into marine conservation planning. Conservation Biology, 35(3): 909–920.
Yu P.C., Matson P.G., Martz T.R., Hofmann G.E. 2011. The ocean acidification seascape and its relationship to the performance of calcifying marine invertebrates: laboratory experiments on the development of urchin larvae framed by environmentally-relevant pCO2/pH. Journal of Experimental Marine Biology and Ecology, 400(1–2): 288–295.
Supplementary material
Supplementary Material 1 (PDF / 1.72 MB).
- Download
- 1.73 MB
Supplementary Material 2 (CSV / 174 KB).
- Download
- 173.05 KB
Information & Authors
Information
Published In
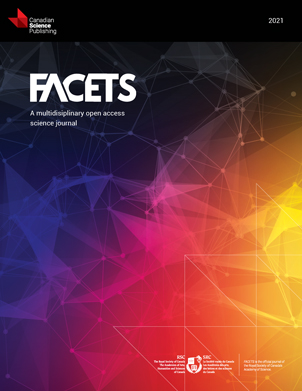
FACETS
Volume 10 • 2025
Pages: 1 - 18
Editor: Dawn Kellett
History
Received: 4 March 2024
Accepted: 28 January 2025
Version of record online: 31 March 2025
Copyright
© 2025 Authors Woodburn, Gregr, Chan, and Stock; and The Crown. This work is licensed under a Creative Commons Attribution 4.0 International License (CC BY 4.0), which permits unrestricted use, distribution, and reproduction in any medium, provided the original author(s) and source are credited.
Data Availability Statement
Data generated or analyzed during this study are provided as supplementary materials.
Key Words
Sections
Subjects
Authors
Author Contributions
Conceptualization: CCM, EJG, KMAC, AS
Data curation: EW
Formal analysis: EW
Funding acquisition: CCM, KMAC, AS
Investigation: EW
Methodology: EW, EJG, AS
Project administration: AS
Software: EJG
Supervision: CCM, KMAC, AS
Visualization: EW, AS
Writing – original draft: EW
Writing – review & editing: CCM, EJG, KMAC, AS
Competing Interests
There are no conflicts of interests.
Funding Information
Natural Sciences and Engineering Research Council of Canada: RGPIN-2020-05032
Canada Research Chairs: CRC-2020-00183
Metrics & Citations
Metrics
Other Metrics
Citations
Cite As
Emie Woodburn, Cathryn C. Murray, Edward J. Gregr, Kai M.A. Chan, and Andy Stock. 2025. The many pathways of climate change affecting coastal ecosystems: a case study of western Vancouver Island, Canada. FACETS.
10: 1-18.
https://doi.org/10.1139/facets-2024-0043
Export Citations
If you have the appropriate software installed, you can download article citation data to the citation manager of your choice. Simply select your manager software from the list below and click Download.
Cited by
1. Challenges in expert ratings of marine habitat and species sensitivity to anthropogenic pressures